Abstract
Water transfers through a multilayered aquifer system are difficult to characterize. This study explores whether the conceptual model of water mixing at depth can be extrapolated over a hydrosystem extended across several tens of kilometers and including multiple aquifer layers. The processes are investigated using a combination of isotope tracers and piezometric monitoring over 10 years. The goal of this approach is to better understand how water transfer occurs throughout a complex and poorly documented hydrosystem of the Mahafaly Plateau in southwestern Madagascar. The results show a clear smoothing of isotopic variability with depth, associated with a smoothing of the recharge peaks. Isotopic values are strongly variable in the near surface (from -6.8 to -2.5‰ 18O) and stabilize at a critical depth (near 20 m) at around -4.7‰ 18O. These results indicate high vertical flows through the aquifer system, where there is neither obvious dominant recharge via preferential pathways nor lateral mixing. Such a strong smoothing effect on groundwater isotopic variability with depth has been rarely observed so clearly over a large spatial scale. These results provide information on a remote groundwater flow system at a scale pertinent to groundwater resource assessment. The results also indicate that the Neogene aquifers of the Mahafaly Plateau are poorly connected with other water resources (rivers, old sedimentary formations) except for the percolation of water towards the deep Eocene karst. This means that groundwater resources in the Ankazomanga Basin are limited and that it is essential to understand and quantify recharge for sustainable groundwater management.
Résumé
Les transferts d’eau dans un système aquifère muti-couches sont difficiles à caractériser. La présente étude examine si le modèle conceptuel de mélange des eaux en profondeur peut être extrapolé à un hydrosystème s’étendant sur plusieurs dizaines de kilomètres et incluant des couches aquifères multiples. Les processus sont explorés en combinant des traçages isotopiques et un contrôle piézométrique sur une période de 10 ans. Le but de cette approche est de mieux comprendre comment le transfert d’eau a lieu à travers l’hydrosystème complexe et peu documenté du Plateau de Mahafaly dans le Sud Ouest de Madagascar. Les résultats montrent un net lissage de la variabilité isotopique avec la profondeur, associé à un lissage des pics de recharge. Les valeurs isotopiques sont fortement variables près de la surface (de -6.8 à -2.5‰ 18O) et se stabilisent à la profondeur critique (près de 20 m) autour de 4.7‰ 18O. Ces résultats indiquent de forts flux verticaux à travers le système aquifère, où il n’y a ni recharge dominante évidente par un cheminement préférentiel ni mélange latéral. Un tel effet de fort lissage sur la variation isotopique des eaux souterraines avec la profondeur a été rarement observé aussi clairement à une échelle spatiale importante. Ces résultats livrent une information sur le système d’écoulement distant des eaux souterraines à une échelle pertinente pour l’évaluation de leur resource. .Les résultats indiquent aussi que les aquifres néogènes du Plateau de Mahafaly sont faiblement connectés à d’autres ressources en eau (rivières, formations sédimentaires anciennes) excepté en ce qui concerne la percolation de l’eau vers l’Eocène karstique profond. Ce qui signifie que les resssources en eaux souterraines du Bassin d’Ankazomanga sont limitées et qu’il est esssentiel de comprendre et de quantifier la recharge en vue d’une gestion durable de ces eaux.
Resumen
Las transferencias de agua a través de un sistema acuífero multicapa son difíciles de caracterizar. Este estudio explora si el modelo conceptual de mezcla de agua en profundidad puede extrapolarse a un sistema hidrológico extendido a lo largo de varias decenas de kilómetros y que incluye múltiples capas acuíferas. Los procesos se investigan utilizando una combinación de trazadores isotópicos y monitoreo piezométrico a lo largo de 10 años. El objetivo de este procedimiento es comprender mejor cómo se produce la transferencia de agua a través de un sistema hidrológico complejo y poco documentado de la meseta de Mahafaly, en el suroeste de Madagascar. Los resultados muestran una evidente atenuación de la variabilidad isotópica con la profundidad, asociada a los picos de recarga. Los valores isotópicos son fuertemente variables en la superficie cercana (de -6.8 a -2.5‰ 18O) y se estabilizan a una profundidad crítica (cerca de 20 m) en torno a -4.7‰ 18O. Estos resultados indican elevados flujos verticales a través del sistema acuífero, donde no hay ni recarga dominante manifiesta a través de trayectorias preferenciales ni mezcla lateral. Rara vez se ha observado tan claramente a gran escala espacial un efecto de atenuación tan fuerte en la variabilidad isotópica de las aguas subterráneas con la profundidad. Estos resultados proporcionan información sobre un sistema remoto de flujo de aguas subterráneas a una escala pertinente para la evaluación de los recursos hídricos subterráneos. Los resultados también indican que los acuíferos neógenos de la meseta de Mahafaly están escasamente conectados con otros recursos hídricos (ríos, formaciones sedimentarias antiguas), excepto por la percolación de agua hacia el karst profundo del Eoceno. Esto significa que los recursos hídricos subterráneos de la cuenca de Ankazomanga son limitados y que es esencial comprender y cuantificar la recarga para una gestión sostenible de las aguas subterráneas.
摘要
水在多层含水层系统中的转移难以表征。该研究探讨了不同深度水混合的概念模型是否可以推广到一个跨越数十公里、包含多个含水层的水系统中。通过在10年间结合同位素示踪剂和压力监测来研究这些过程。该方法的目标是更好地理解马达加斯加西南部Mahafaly高原复杂且缺乏详细资料的水系统中水的转移过程。研究结果显示同位素变化随着深度的增加呈现明显的平滑趋势,与此同时补给峰值也平滑。近地表处同位素值变化很大(从-6.8‰到-2.5‰的18O),在临近20米的关键深度稳定在约-4.7‰的18O。这些结果表明含水层系统中存在很强的垂直流动,既没有明显的优势通路补给,也没有侧向混合。在大范围的空间尺度上很少观察到如此明显的地下水同位素变化随深度平滑的现象。这些结果提供了有关马达加斯加西南部马哈法利高原遥远地下水流系统的信息,这对于地下水资源评估具有重要意义。结果还表明,Mahafaly高原上第三系含水层与其他水资源(河流、古老的沉积层)联系较差,除了水向深部始新世岩溶层渗漏外。这意味着Ankazomanga盆地的地下水资源有限,并且必须了解和量化补给过程以实现可持续的地下水管理。
Resumo
As transferências de água através de um sistema aquífero multicamadas são difíceis de caracterizar. Este estudo explora se o modelo conceitual de mistura de água em profundidade pode ser extrapolado para um hidrossistema que se estende por várias dezenas de quilômetros e inclui aquíferos multicamadas. Os processos são investigados usando uma combinação de traçadores de isótopos e monitoramento piezométrico ao longo de 10 anos. O objetivo desta abordagem é compreender melhor a transferência de água através de um hidrossistema complexo e pouco documentado do Planalto Mahafaly, no sudoeste de Madagascar. Os resultados mostram uma clara suavização da variabilidade isotópica em profundidade, associada a uma suavização dos picos de recarga. Os valores isotópicos são fortemente variáveis na superfície próxima (de -6.8 a -2.5‰ 18O) e se estabilizam em uma profundidade crítica (perto de 20 m) em torno de -4.7‰ 18O. Esses resultados indicam elevados fluxos verticais através do sistema aquífero, onde não há nem recarga dominante óbvia via caminhos preferenciais nem mistura lateral. Esse forte efeito de suavização na variabilidade isotópica das águas subterrâneas com a profundidade raramente foi observado tão claramente em grande escala espacial. Esses resultados fornecem informações sobre um sistema remoto de fluxo de águas subterrâneas em uma escala pertinente à avaliação de recursos de águas subterrâneas. Os resultados também indicam que os aquíferos neógenos do Planalto Mahafaly são pouco conectados com outros recursos hídricos (rios, antigas formações sedimentares), exceto pela percolação da água em direção ao carste profundo do Eoceno. Isso significa que os recursos hídricos subterrâneos na Bacia Ankazomanga são limitados e que é essencial entender e quantificar a recarga para o gerenciamento sustentável das águas subterrâneas.
Similar content being viewed by others
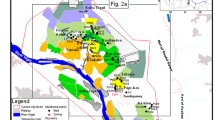
Introduction
Stable isotope (δ18O and δ2H) values of precipitation are highly variable, both spatially and temporally (Dansgaard 1964; Rozanski et al. 1992). The temporal variability can be observed at the seasonal scale and the rainfall event scale (Munksgaard et al. 2012). This variability in rainfall isotope values entering groundwater systems can result in high variability of stable isotope values in near-surface groundwater. Moreover, near-surface evaporation processes and recharge by open water surfaces add other sources of isotope signal variability (Benettin et al. 2018). Within deeper aquifer systems, the stable isotope values are less variable due to mixing. Where vertical groundwater flow is dominant, the recharging waters from different rainfall events are progressively mixed resulting in a less variable isotopic signature with depth. As a result, deep groundwater where vertical flow is dominant can have an isotopic signal close to the average value of the precipitation waters recharging the system over multiple time scales (Fig. 1).
Darling and Bath (1988) highlight the decrease of temporal variations of δ18O of water in a lysimeter of 5 m depth. Eichinger et al. (1984) and Beyer et al. (2018) confirmed this attenuation of isotopic variability in natural soils and the vadose zone. Several studies show the attenuation of seasonal variability in groundwater isotope signals for various geological contexts (e.g. Hamid et al. 1989; Clark and Fritz, 1997; Schwarz et al. 2009). Clark and Fritz (1997) assumed that the depth without seasonal variation (or mixing depth) depends on the physical properties of the sub-surface lithology. Wright and Novakowski (2019) also reported that variations in the mixing depth were related to changes in the geological context (sandstone, limestone, and crystalline). This mixing depth is also often situated below the water table (Clark and Fritz, 1997). For example, Petrella and Celico (2013) conducted a 14-month study of water isotopes at multiple depths in a single borehole in a karst environment, which identified a mixing depth around 15 m below the water table.
The vertical water mixing with depth can be disrupted in two cases: i) if lateral or deep groundwater inflows with a different isotopic signature disturb the mixing process. Several studies conducted in multilayer aquifers have highlighted isotopic signals specific to each groundwater level due to different recharge conditions (Cartwright and Weaver 2005; Filippini et al., 2015; Pilla et al. 2006, Qian et al., 2013). The isotopic composition of groundwater in the intermediate aquifer is thus a mixing between different end-members. ii) If waters are recharged via preferential pathways and thus bypass the vertical mixing process. Brooks et al. (2010) showed that preferential flows towards depth occur depending on the soil water status. Faults or groundwater pumping can create unexpected preferential connections to upper or lower aquifers that short-circuit the normal slow transfer and mixing processes expected (Hamed et al. 2011; Dassi, 2011; Tarki et al., 2016). Cruz Jr et al. (2005) showed that in karst systems in Brazil, preferential flows, via fractures and conduits, disrupt the organization of the isotopic signal too much to determine a mixing depth.
This article investigates if the conceptual model of water mixing with depth can be extended to the scale of a hydrosystem of several tens of kilometers wide, and includes multilayer aquifers. The complex hydrosystem of the Mahafaly Plateau, in southwestern Madagascar, is a poorly documented area lacking geological and hydrogeological information, and this limits the sustainable management of water resources. Therefore, this study aims to improve the understanding of groundwater flows through this multilayered aquifer system using isotope and piezometric monitoring.
Materials and methods
Geographic and climatic settings
The study area is located in southwest Madagascar in the southeast of the city of Toliara. Onilahy River, in the North, is the only perennial stream in the region. The Mozambique Channel borders the study area to the west (Fig. 2a). The rural study area is very isolated and these characteristics impose strong logistical constraints for any hydrogeological investigation.
Southwest Madagascar is a semi-arid region and the driest area of the country. Annual rainfall is on average 400 mm near the coast with slightly increasing rainfall inland. In comparison, the eastern coast of Madagascar is amongst the wettest part of the island with more than 2000 mm/year (Ollivier et al. 2023). The wet season occurs from December to March, and the dry season occurs from April to November. Most of the wet season precipitation occurs during convective events, inducing strong spatial and temporal irregularities in rainfall. Heavy rains are mostly generated by cyclones and tropical storms. These heavy rainfalls usually originate from the Indian Ocean (East), but there are several recent examples of cyclones originating from the Mozambique Channel (West). The Mahafaly Plateau is a flat area (Fig. 2). Therefore, it is assumed that the altitudinal isotopic gradient (Dansgaard, 1964) is negligible across the study area. The Ankazomanga Basin is the area studied further in this work (orange area in Figure 2). This area is located on the Mahafaly Plateau between elevations 170 and 250 meters above sea level (m.a.s.l).
Geological and hydrogeological settings
The study area is located on recent sedimentary rocks (Mesozoic and Cenozoic). There are Cretaceous rock outcrops to the East of the study area (Fig. 2b). The Mahafaly Plateau is composed of an ensemble of Eocene carbonate rocks described in detail by Karche (1961). These Eocene formations were divided into three units by Aurouze (1957): i) Eocene 1 (E1) composed of nummulitic limestone, calcareous sandstone, and oolitic limestone; ii) Eocene 2 (E2) composed of clayey limestone with orbitolinids; and iii) Eocene 3 (E3) composed of marly limestone, oyster marl, and gypsum marl.
These Eocene formations are karstified with an advanced degree of alteration depending on the facies. Unit E1 is the most karstified unit and contains many spectacular sinkholes and a highly developed cavities network (André et al. 2005). Consequently, the Eocene carbonate formations are complex from a hydrogeological point of view due to this heterogeneity.
The composition of the Ankazomanga basin (orange zone in Fig. 2a) is Continental Neogene formations with alternations by several types of sandstone, marl, sand and clay. The Continental Neogene formations do not outcrop in the Ankazomanga basin because the area is entirely covered by alterites (called red sand). It is assumed that these red sands play a buffering and homogenizing role for infiltrated water. The sand cover makes the geological characterization of the study area difficult. Carrière et al. (2018) explored the Ankazomanga basin using geophysical and hydrogeological approaches. The results emphasized the strong heterogeneity of the Neogene Continental formations (Fig. 3b). Indeed, the conductive clay formations, shown in blue in Figure 3b, are discontinuous and non-tabular. Two boreholes drilled as part of the same study (Fig. 3c) confirm the variability of Neogene facies. It is not possible to establish any link between two boreholes separated by less than 2 km (Fig. 3c). Consequently, the Continental Neogene formations are difficult to characterize in terms of groundwater flow processes. These formations constitute a complex multilayered aquifer perched above the karst developed in Eocene rocks (Carrière et al. 2018).
Geophysical and drilling investigation around Tokoendolo village (Carrière et al. 2018). (a) Location map of Tokoendolo village and positioning of the geophysical transect. (b) Geophysical cross-section obtained with time domain electromagnetic (TDEM) soundings with 50×50 m loop. (c) Borehole logs in Continental Neogene formations with a simplified representation of colors observed on cuttings
Carrière et al. (2018) produced a piezometric map (Fig S1 in the electronic supplementary material (ESM)) showing that groundwater flows through the Continental Neogene aquifer from east to west. There is no known aquitard between the Neogene and Eocene. Given the recharge and discharge dynamics observed in the Neogene aquifers (Carrière et al. 2021), it can be assumed that water from the Neogene aquifers percolates into the Eocene karst.
Hydrochemical monitoring highlighted that the Eocene karst and Continental Neogene waters are clearly contrasted. The analysis of Na vs. Cl (Fig. S2 in the ESM) shows that the Ankazomanga basin waters (Continental Neogene) have very low mineralization, with less than 3 meq/L of Na and Cl. However, the coastal springs waters (Eocene karst) are highly mineralized with 20 to 30 meq/L of Na and Cl. This high mineralization is related to saline intrusions that can propagate far inland due to the high permeability of karst (Guyot 2002).
Stable isotopes of waters
Groundwater was collected at 46 locations from two areas: i) multilayer Neogene aquifers within the Ankazomanga basin on the Mahafaly Plateau. Samples were taken from 38 traditional wells (Fig. 4a), cemented wells (Fig. 4b), and modern boreholes (Fig. 4c) with depths ranging from 3 to 45 m (blue symbols in Fig. 2a). ii) the deep karst developed in Eocene rocks were sampled in 3 sinkholes, 5 coastal springs and 2 cemented wells. These waters represent the natural outlet of the karst system (green symbols in Fig. 2a). The water level in the karst is close to sea level according to the Chevron Oil borehole drilled in 1972 at 40 km from the coast (Guyot 2002). Considering that the Ankazomanga basin is located 160 to 250 m above sea level, the depth of karst water is assumed to be lower than 100 m deep in the study area.
A total of 119 samples were collected over 10 campaigns carried out between 2013 and 2022 during both dry and wet periods. Data and well specifications are summarized in Table S1 in the ESM. The sampled wells were exploited, avoiding problems related to water stagnation. Only 15 points were sampled several times to produce a monitoring. Groundwater was collected in smoked glass vials. Samples were stored in locations protected from temperature variations and then placed in the refrigerator as soon as possible.
Water was analyzed on a Los Gatos isotope ratio infrared spectrometer (IRIS) at the University of Avignon in France (LGR DLT-100 liquid water stable analyzer accuracy ±0.2‰ vs. VSMOW for δ18O and ±1‰ vs. VSMOW for δ2H).
Piezometry monitoring
Piezometry monitoring started in 2014 as part of the groundwater resource observatory for southwestern Madagascar (GROSoM), (Carrière et al. 2021). On the Mahafaly Plateau, six wells of the multilayer Neogene aquifer are still monitored in the Ankazomanga basin. They have been equipped with automatic piezometric probes (model TD-DIVER) allowing an accuracy of ± 1 cm H2O. Two barometric probes (Baro-DIVER, accuracy ± 0.5 cm H2O) are also used for the compensation of piezometric data. The equipment was programmed for hourly data acquisition.
The probes were installed in wells with depths ranging from 8 to 35 m. For each well, the measurements provided by the automatic probes were verified with a water level meter both during the installation of the sensors and a few months later for calibration control.
Results and interpretation
Stable isotope (δ18O vs δ2H) results show a large dispersion of δ18O values (-7 and -2 ‰) and δ2H values (-50 and -5 ‰; Fig. 5). Groundwater points lie slightly to the right of the global meteoric water line (GMWL), which is likely a result of local rainfall variations (local rainfall isotope values were not available to plot a local meteoric water line). Only one groundwater sample, from the Ankandy shallow well (5 m), shows a deviation to the right of the GMWL that may indicate the effects of evaporation on groundwater, however a local meteoric water line is required to confirm this. Groundwater isotope values from both modern and traditional well points on the Mahafaly Plateau are widely scattered. The isotopic values from six wells at various depths were identified and are plotted with individual symbols in Fig. 5. This highlights that wells shallower than 10 m (Ankandy and Ankazomanga_P01) have isotopic values that show a greater temporal variability (-7 to -3‰ for δ18O) compared with the deeper wells (> 10 m) with stable isotope values ranging from -5 to -4.5‰ for δ18O. The karst groundwater points are grouped in the center of the scatterplot. This leads one to assume that various mixing and homogenization processes occur to smooth the isotopic signal across the multilayer Neogene aquifer and the Eocene karst.
Dual isotope plot of groundwater sampled in the Ankazomanga basin and coastal springs at the outlet of the karst system. Signals of 6 wells in the Ankazomanga basin are presented individually to appreciate the signal variability between campaigns (Ankandy, Ankazomanga_P01, Ankazomanga_P06, Maroarivo, Ambatofotsy, Vovomena). The data were acquired during 10 sampling campaigns conducted between 2013 and 2022. GMWL is the global meteoric water line determined by Rozansky et al. (1993). VSMOW is the Vienna standard mean ocean water
When the isotopic signal is plotted as a function of water depth, it highlights the larger variability between the isotopic signal of shallow groundwaters (< 10 m) compared with deeper (> 10 m) groundwater for both δ2H and δ18O (Fig. 6). This signal heterogeneity can be explained by both spatial and temporal variability in the water recharging the hydrosystem. The temporal variability is likely due to the isotope variability of the event and seasonal-scale rainfall recharging the aquifers. The spatial variability between wells is likely due to variations in infiltration conditions due to the heterogeneous lithology (e.g. Fig. 3) influencing the recharge rates of rainfall events and subsurface mixing processes. With depth, the recharging waters are mixed and provide an isotopic signature representative of the longer-term recharge average. For example, the Vovomena well taps groundwater at 28 m depth in the Continental Neogene aquifer system and shows a very stable signal over the three sampling campaigns (average of -4.85‰ for δ18O and -32.6‰ for δ2H). In addition, the signal of the Vovomena well is very close to the karst groundwater values (average of -4.91‰ for δ18O and -33.4‰ for δ2H). The scatterplot has the shape of a symmetrical funnel (Fig. 6). This indicates that there is no significant water input at depth with a contrasting signal.
Variability of groundwater isotopic signatures with depth: (a) δ18O and (b) δ2H. Groundwater was sampled in the Ankazomanga basin and coastal springs at the outlet of the karst system. Signals of 6 wells in the Ankazomanga basin are presented individually to appreciate the signal variability between campaigns (Ankandy, Ankazomanga_P01, Ankazomanga_P06, Maroarivo, Ambatofotsy, Vovomena). The data result from 10 sampling campaigns conducted between 2013 and 2022. VSMOW is the Vienna standard mean ocean water
The piezometric time series of wells monitored in the Ankazomanga basin show seasonal dynamics of aquifer recharge and discharge. The recharge peak is reached towards the end of the rainy season around March. The well exploitation causes a drop in the piezometric level, which is visible with daily frequency, especially during dry period. It is assumed that withdrawals may have a slight tendency to attenuate seasonal recharge. The variability of recharge dynamics between wells agreed with the hypothesis of water mixing with depth. Figure 7 displays the piezometric dynamics during the 2016/2017 recharge period. This is a typical year when recharge is strong enough to be visible. Unfortunately, this is the only year for which one can compare all six piezometers simultaneously. Indeed, field conditions are demanding for the equipment and frequent deteriorations occur and cause gaps in piezometric records. The full-time series are presented in Fig S3 in the ESM.
Time series 2016/2017 of daily rainfall at Ankazomanga village and six piezometers within the Ankazomanga basin organized by increasing depth. The depths and altitudes of inflow are indicated in the upper left corner of each graph (e.g. Ankandy depth is 5m). The measurement time step of the piezometry is 1 h. The drops in piezometry during the day are related to water uptakes (m.a.s.l. is meters above sea level)
Wells tapping the shallowest water (Ankandy and Ankazomanga_P01) are the most reactive to the rain (Fig. 7). Their piezometric levels increase abruptly with heavy rains. As soon as the rains end, their piezometric level begins a long decline. As the wells tap deeper groundwater, recharge peaks occur more smoothly. A delay of several months is noticeable between the rainy season and the recharge peak on these deep wells. These delays are explained by the time necessary for water infiltration toward the water table. This delay is particularly long for the Ankazomanga_P06 well. This is due to a complementary lateral water movement in addition to the vertical water transfer; this borehole is located in a zone where the bottom of the aquifer is lower (Carrière et al. 2018). The Vovomena borehole has a fairly constant water level over the year and recharge occurs as a dispersed, low-intensity pulse. This borehole has low productivity (0.3 m3/h). Consequently, this borehole is rapidly dewatered by exploitation but it does not dry up even during the dry season.
Discussion
Isotopic and piezometric data provide consistent information for the Mahafaly Plateau. Isotope tracing highlights a smoothing of the signal variability with depth without disturbance by other water supplies with a different isotopic signal. This characteristic is typical of high vertical flows (Clark 2015). The piezometry highlights a smoothing of recharge peaks with depth. This information allows one to better understand the main hydrogeological functions of the Mahafaly Plateau.
These results suggest that vertical transfers and the associated mixing process are not (or rarely) disturbed by another source of water (i.e. lateral recharge or surface water entering via preferential pathways). In other studies of multilayer aquifers, the presence of lateral flows or preferential recharge to deep aquifers has been identified to disturb the homogenizing effect on isotopic signals (Cartwright and Weaver 2005; Pilla et al. 2006, Qian et al., 2013; Filippini et al., 2015).
A critical mixing depth of about 20 m is observed if one refers to the definition of Clark and Fritz (1997). Those authors defined that the critical depth was reached at the depth where all the points are centered on the mean plus or minus the standard deviation. However, one must remain cautious about the precision of this depth, as this study only has 4 boreholes of more than 20 m. This critical mixing depth is consistent with that found by Petrella and Cecilo (2013) who found 15 m in a karst in Italy.
The clear mixing process with depth is explained by the topographic and geological configuration of the Mahafaly Plateau. The absence of uneven topography favours a homogeneous infiltration of water towards deeper aquifers. The Mahafaly Plateau is a flat area where the altitudinal isotopic gradient is negligible. There is therefore no disturbance related to differences in precipitation altitude. The Continental Neogene formations play a buffering and homogenizing role for infiltrated water.
The analysis of relationships between hydrochemical parameters and depth shows a smoothing effect with depth. This concerns some variables such as fluorine (F), potassium (K) and chlorine/bromine ratio (Cl/Br) but this is less clear than for water isotopes (Fig. S4 in the ESM). This less relevance of hydrochemical variables to demonstrate the mixing effect of water with depth is also related to the multiple possible interactions between water and rocks that make the relationship more complex.
The groundwater isotopic composition of karstified Eocene aquifers is equal to the mean value of the near-surface aquifers observed in the Neogene. This is coherent with the expected mixing process from Neogene to Eocene. The isotopic composition of deep karst should be a mix between the unconfined part of the Eocene karst and the part covered by the Continental Neogene. It is assumed that the mean isotopic signal of rainfall in the Ankazomanga basin is similar to the signal of the whole Mahafaly karst Plateau. There is also a strong smoothing effect occurring within the unconfined part of the karst aquifer.
In terms of water resource management, these results support the hypothesis that the Continental Neogene aquifers of the Ankazomanga basin are fairly isolated from other water resources (river, old sedimentary formations, deep Eocene karst). It can only be assumed that there are vertical transfers of water from the Neogene to the deep karst. The Neogene formations form a perched aquifer that is only recharged by rainfall. This means that understanding and quantifying rainfall recharge is essential to promote sustainable water resource management. For example, the activities of several development actors (i.e. NGOs, UN agencies) are promoting the emergence of irrigated market gardening. This commendable activity, which is a source of food diversification, must be done without the risk of over-exploitation of underground resources. For this reason, the GROSoM observatory (Carrière et al. 2021) has been set up since 2014 to monitor long-term piezometric dynamics.
Conclusions and perspectives
This study evaluated whether the conceptual model of smoothing water isotope signals with depth can be extended over an hydrosystem extended across several tens of kilometers including multiple aquifer layers. Other authors have tested this concept at smaller scales: i) over the root zone (Beyer et al. 2018), ii) in the unsaturated zone (Schwarz et al. 2009), and iii) in the saturated zone (Petrella and Celico 2013). This work demonstrated that the smoothing effect of water isotopic signals with depth can be observed on a larger scale. Moreover, this is consistent with the piezometric time series where a smoothing effect of the recharge peak with depth is observed.
This simplified view of water transfer organization in the underground allows a better understanding of the hydrogeological functioning. The Mahafaly Plateau transfers are essentially vertical. There is no significant lateral recharge with a contrasted isotopic signature from the Onilahy River to the North or from old sedimentary aquifers to the East. No preferential infiltration is identified because the omnipresent red sand cover in the Ankazomanga basin provides a buffer effect for water transfer. No contamination is detected from superficial evaporated water. The Continental Neogene of the Ankazomanga basin constitutes a multi-layered aquifer system that is fairly isolated from other water resources.
For further interpretation it would be relevant to: i) Estimate residence time in groundwater. ii) Monitor the isotopic quality of rainwater during one or more hydrological cycles to determine the local meteoric water line. This action has not been carried out until now due to the access difficulties of the area. However, it would allow one to know the isotopic signal entering the hydrosystem to improve hydrogeological functioning understanding. iii) Carry out bacteriological monitoring of the groundwater. This will allow one to verify if the mixing process occurs progressively with depth or if preferential pathways make possible the penetration of polluted surface water directly into the deeper aquifers.
References
André G, Bergeron G, Guyot L (2005) Contrôle structural et tectonique sur l’hydrogéologie karstique du plateau Mahafaly (domaine littoral semi-aride, sud-ouest de Madagascar) (Structural and tectonic control on the karst hydrogeology of the Mahafaly Plateau (semi-arid coastal domain, southwest of Madagascar)). Karstologia 45–46:29–40
Aurouze J (1957) Carte et notice hydrogéologique du Sud de Madagascar (1/500000). Service Géologique de Madagascar, Antananarivo
Benettin P, Volkmann TH, von Freyberg J, Frentress J, Penna D, Dawson TE, Kirchner JW (2018) Effects of climatic seasonality on the isotopic composition of evaporating soil waters. Hydrology and Earth System Sciences 22:2881–2890. https://doi.org/10.5194/hess-22-2881-2018
Beyer M, Hamutoko J, Wanke H, Gaj M, Koeniger P (2018) Examination of deep root water uptake using anomalies of soil water stable isotopes, depth-controlled isotopic labeling and mixing models. Journal of Hydrology 566:122–136. https://doi.org/10.1016/j.jhydrol.2018.08.060
Brooks JR, Barnard HR, Coulombe R, McDonnell JJ (2010) Ecohydrologic separation of water between trees and streams in a Mediterranean climate. Nature Geoscience 3:100. https://doi.org/10.1038/NGEO722
Carrière SD, Chalikakis K, Ollivier C, Heath T, Mangin M, Kempf J, Randriatsitohaina JB, Gillon M, Razakamanana T, Lapègue J (2018) Sustainable groundwater resources exploration and management in a complex geological setting as part of a humanitarian project (Mahafaly Plateau, Madagascar). Environmental earth sciences 77:734. https://doi.org/10.1007/s12665-018-7909-1
Carrière SD, Health T, Rakotomandrindra PF, Ollivier C, Rajaomahefasoa RE, Rakoto HA, Lapègue J, Rakotoarison YE, Mangin M, Kempf J, Razakamanana T, Chalikakis K (2021) Long-term Groundwater Resource Observatory for Southwestern Madagascar (GROSoM). Hydrological Processes e14108. https://doi.org/10.1002/hyp.14108
Cartwright I, Weaver TR (2005) Hydrogeochemistry of the Goulburn Valley region of the Murray Basin, Australia: implications for flow paths and resource vulnerability. Hydrogeology Journal 13:752–770. https://doi.org/10.1007/s10040-003-0318-9
Clark I (2015) Groundwater geochemistry and isotopes. CRC pressTaylor & Francis Group. https://doi.org/10.1201/b18347
Clark I, Fritz P (1997) Groundwater, Chapter 4. In: Environmental isotopes in Hydrogeology, First edn. Lewis Publishers Boca Raton, New-York, pp 79–110
Cruz FW Jr, Karmann I, Viana O Jr, Burns SJ, Ferrari JA, Vuille M, Sial AN, Moreira MZ (2005) Stable isotope study of cave percolation waters in subtropical Brazil: Implications for paleoclimate inferences from speleothems. Chemical Geology 220:245–262. https://doi.org/10.1016/j.chemgeo.2005.04.001
Dansgaard W (1964) Stable isotopes in precipitation. Tellus 16:436–468. https://doi.org/10.1111/j.2153-3490.1964.tb00181.x
Darling W, Bath A (1988) A stable isotope study of recharge processes in the English Chalk. Journal of Hydrology 101:31–46. https://doi.org/10.1016/0022-1694(88)90026-1
Dassi L (2011) Investigation by multivariate analysis of groundwater composition in a multilayer aquifer system from North Africa: A multi-tracer approach. Applied Geochemistry 26:1386–1398. https://doi.org/10.1016/j.apgeochem.2011.05.012
Eichinger L, Merkel B, Nemeth G, Salvamoser J, Stichler W, Udluft P (1984) Seepage velocity determinations in unsaturated quaternary gravel. Recent investigations in the zone of aeration, symposium proceedings, Munich. pp 303–313
Filippini M, Stumpp C, Nijenhuis I, Richnow HH, Gargini A (2015) Evaluation of aquifer recharge and vulnerability in an alluvial lowland using environmental tracers. Journal of Hydrology 529:1657–1668. https://doi.org/10.1016/j.jhydrol.2015.07.055
Guyot L (2002) Reconnaissance hydrogéologique pour l’alimentation en eau d’une plaine littorale en milieu semi-aride : Sud-Ouest de Madagascar. Université de Nantes
Hamed Y, Dassi L, Tarki M, Ahmadi R, Mehdi K, Dhia HB (2011) Groundwater origins and mixing pattern in the multilayer aquifer system of the Gafsa-south mining district: a chemical and isotopic approach. Environ Earth Sci 63:1355–1368. https://doi.org/10.1007/s12665-010-0806-x
Hamid S, Dray M, Ferhi A, Dorioz JM, Normand M, Fontes JC (1989) Etude des transferts d’eau à l’intérieur d’une formation morainique dans le bassin du Léman-Transferts d’eau dans la zone non saturée. Journal of Hydrology 109:369–385. https://doi.org/10.1016/0022-1694(89)90025-5
Karche J-P (1961) Contribution a l’étude géologique et hydrogéologique du plateau calcaire Mahafaly et aperçu sur l’hydrogéologie de Madagascar. Université de Paris
Munksgaard NC, Wurster CM, Bass A, Bird MI (2012) Extreme short-term stable isotope variability revealed by continuous rainwater analysis. Hydrological Processes 26:3630–3634. https://doi.org/10.1002/hyp.9505
Ollivier CC, Carrière SD, Heath T, Olioso A, Rabefitia Z, Rakoto H, Oudin L, Satgé F (2023) Ensemble precipitation estimates based on an assessment of 21 gridded precipitation datasets to improve precipitation estimations across Madagascar. Journal of Hydrology: Regional Studies 47:101400. https://doi.org/10.1016/j.ejrh.2023.101400
Petrella E, Celico F (2013) Mixing of water in a carbonate aquifer, southern Italy, analysed through stable isotope investigations. International Journal of Speleology 42:4. https://doi.org/10.5038/1827-806X.42.1.4
Pilla G, Sacchi E, Zuppi G, Braga G, Ciancetti G (2006) Hydrochemistry and isotope geochemistry as tools for groundwater hydrodynamic investigation in multilayer aquifers: a case study from Lomellina, Po plain, South-Western Lombardy, Italy. Hydrogeol J 14:795–808. https://doi.org/10.1007/s10040-005-0465-2
Qian H, Li P, Wu J, Zhou Y (2013) Isotopic characteristics of precipitation, surface and ground waters in the Yinchuan plain, Northwest China. Environ Earth Sci 70:57–70. https://doi.org/10.1007/s12665-012-2103-3
Rozanski K, Araguás-Araguás L, Gonfiantini R (1992) Relation Between Long-Term Trends of Oxygen-18 Isotope Composition of Precipitation and Climate. Science, New Series 258:981–985. https://doi.org/10.1126/science.258.5084.981
Schwarz K, Barth J, Postigo-Rebollo C, Grathwohl P (2009) Mixing and transport of water in a karst catchment: a case study from precipitation via seepage to the spring. Hydrology and Earth System Sciences 13:285–292. https://doi.org/10.5194/hess-13-285-2009
Tarki M, Ben Hammadi M, El Mejri H, Dassi L (2016) Assessment of hydrochemical processes and groundwater hydrodynamics in a multilayer aquifer system under long-term irrigation condition: A case study of Nefzaoua basin, southern Tunisia. Applied Radiation and Isotopes 110:138–149. https://doi.org/10.1016/j.apradiso.2016.01.009
Wright SN, Novakowski KS (2019) Groundwater recharge, flow and stable isotope attenuation in sedimentary and crystalline fractured rocks: Spatiotemporal monitoring from multi-level wells. Journal of Hydrology 571:178–192. https://doi.org/10.1016/j.jhydrol.2019.01.028
Acknowledgements
The authors would like to express their gratitude to: the funding organizations (Sustainable Initiatives for Water (SIWA), DG International Cooperation and Development (DEVCO)); the local partners (the Malagasy Water Direction (DREAH), the Malagasy Meteorological Direction (SMR)); the AAH members who work on this project (in particular Rindra Hagamamy Rakotojoelimaria, Tsitola Benahy Ramananjato); Milanka Babic, Julien Dupont, and Roland Simler for water analysis; and Gaspard Magniny for the last sampling campaign.
Author information
Authors and Affiliations
Corresponding author
Ethics declarations
Conflict of interest
On behalf of all authors, the corresponding author states that there is no conflict of interest.
Additional information
Publisher’s note
Springer Nature remains neutral with regard to jurisdictional claims in published maps and institutional affiliations.
Supplementary information
ESM 1
(PDF 1122 kb)
Rights and permissions
Open Access This article is licensed under a Creative Commons Attribution 4.0 International License, which permits use, sharing, adaptation, distribution and reproduction in any medium or format, as long as you give appropriate credit to the original author(s) and the source, provide a link to the Creative Commons licence, and indicate if changes were made. The images or other third party material in this article are included in the article's Creative Commons licence, unless indicated otherwise in a credit line to the material. If material is not included in the article's Creative Commons licence and your intended use is not permitted by statutory regulation or exceeds the permitted use, you will need to obtain permission directly from the copyright holder. To view a copy of this licence, visit http://creativecommons.org/licenses/by/4.0/.
About this article
Cite this article
Carrière, S.D., Rakotomandrindra, P.F.M., Heath, T. et al. Water mixing processes in a complex multi-layer hydrosystem in southwestern Madagascar: a combined isotopic and piezometry approach. Hydrogeol J 31, 2337–2346 (2023). https://doi.org/10.1007/s10040-023-02731-5
Received:
Accepted:
Published:
Issue Date:
DOI: https://doi.org/10.1007/s10040-023-02731-5