Pesticides and the Microbiome-Gut-Brain Axis: Convergent Pathways in the Pathogenesis of Parkinson’s Disease
Abstract
The increasing global burden of Parkinson’s disease (PD), termed the PD pandemic, is exceeding expectations related purely to population aging and is likely driven in part by lifestyle changes and environmental factors. Pesticides are well recognized risk factors for PD, supported by both epidemiological and experimental evidence, with multiple detrimental effects beyond dopaminergic neuron damage alone. The microbiome-gut-brain axis has gained much attention in recent years and is considered to be a significant contributor and driver of PD pathogenesis. In this narrative review, we first focus on how both pesticides and the microbiome may influence PD initiation and progression independently, describing pesticide-related central and peripheral neurotoxicity and microbiome-related local and systemic effects due to dysbiosis and microbial metabolites. We then depict the bidirectional interplay between pesticides and the microbiome in the context of PD, synthesizing current knowledge about pesticide-induced dysbiosis, microbiome-mediated alterations in pesticide availability, metabolism and toxicity, and complex systemic pesticide-microbiome-host interactions related to inflammatory and metabolic pathways, insulin resistance and other mechanisms. An overview of the unknowns follows, and the role of pesticide-microbiome interactions in the proposed body-/brain-first phenotypes of PD, the complexity of environmental exposures and gene-environment interactions is discussed. The final part deals with possible further steps for translation, consisting of recommendations on future pesticide use and research as well as an outline of promising preventive/therapeutic approaches targeted on strengthening or restoring a healthy gut microbiome, closing with a summary of current gaps and future perspectives in the field.
INTRODUCTION –THE INCREASING GLOBAL BURDEN OF PARKINSON’S DISEASE AND POSSIBLE LINKS TO ENVIRONMENTAL EXPOSURES
Parkinson’s disease (PD) is the second-most common neurodegenerative disorder, affecting 2–3% of the population≥65 years of age. While we still lack a comprehensive understanding of the etiology, the underlying α-synuclein (α-syn) pathology and (neuro)inflammation are widely recognized as key players driving PD progression [1], although other factors, such as tau pathology, are likely important to a lesser extent [2, 3]. Among neurological disorders, PD has undergone the fastest growth in prevalence and disability in recent years and has become one of the leading causes of disability worldwide [4, 5]. According to data obtained from the Global Burden of Disease 2019, increasing trends of PD burden were observed globally in most regions and countries [6]. This allows us to talk about a Parkinson’s pandemic [7] similar to the pandemic of other non-communicable diseases—fueled by social, political, economic and environmental trends and changes [8]. The aging of the population contributed substantially to the rise in the global prevalent number of PD cases from 1990 (2.5 million) to 2019 (8.5 million), but an upward trend is also evident in the age-standardized rate of prevalence and incidence, indicating that global aging may not be the only reason for the increased prevalence, and other factors are likely important [6]. Although socioeconomic level is, in general, positively associated with health [9], the opposite seems to be true for PD, with age-standardized disability-adjusted life-years due to PD being increased with higher sociodemographic index (SDI) [5]. This, on the one hand, may be related to longer survival of more wealthy PD patients due to more sophisticated and also more expensive treatment options. On the other hand, growing world industrialization, pronounced in countries with higher SDI, may be linked to several environmental factors driving the increased global burden of PD, such as exposure to pesticides [10], solvents [10] or metals [11, 12]. The largest increase in the age-adjusted prevalence rate of PD between 1990 and 2016 worldwide was documented in China, a middle SDI country that has undergone rapid industrial growth since 1990 [5]. Occupational exposure and industrial pollution also accelerated the increasing trends in low and middle SDI areas [6, 11, 13, 14]. These factors are particularly interesting, as they represent a major challenge to public health as well as an open door for risk modification on a large scale.
The association of pesticides exposure and risk of PD is well known [10, 15, 16], being included as an independent risk factor for PD in the original and updated version of the International Parkinson and Movement Disorders Society (MDS) research criteria for prodromal PD (positive likelihood ratio of 1.5) [17, 18]. Based on several meta-analyses [10, 19, 20] and expert reviews, PD was recognized as an occupational disease in agriculture professionals in France in 2012 (under the conditions of a diagnosis confirmed by a neurologist and professional exposure to pesticides for 10 years or more) [21]. The epidemiological evidence for a link between PD and pesticides is strong, yet the global use of pesticides is still at or near its highest levels, including those with a clear impact on PD pathogenesis, such as paraquat. Some measures have been taken, and paraquat has been gradually banned in almost 60 countries, including Switzerland (1989), the United Kingdom, the European Union (2007) [22], South Korea (2011) [23], China (2016) [24] or lately Brazil and Taiwan (2020). However, the export to other countries, including African countries, India, Australia, or the United States, continues [7].
Despite the already established connection between pesticides and PD, the exact mechanisms of how pesticides contribute to PD pathology are still under investigation. While direct effects of pesticides on the central nervous system (CNS) have been extensively investigated, especially in the mouse model of PD, effects on the peripheral (PNS) and the enteric nervous system (ENS) are not fully understood yet. The microbiome-gut-brain axis, the bidirectional communication between the ENS and the CNS, including the vagus nerve, endocrine as well as immune pathways and bacterial metabolites, has been in the scope of PD research in recent years [25–27]. In this regard major focus has been placed on the influence of nutrition, pre- and probiotics or fecal microbiota transplantation (FMT) on the microbiome with regard to possible future treatment strategies [28]. However, the impact of pesticides on the microbiota has started to gain more attention only recently, since they may have substantial detrimental effects on microbiome composition and function, which may play an important role in the pathophysiology of PD and other neurodegenerative and non-communicable diseases [29–32].
We acknowledge the tremendous work already done in this area of research, as represented by multiple reviews targeting the topics of environmental exposures and risk of PD, as well as the role of the microbiome-gut-brain axis in the pathogenesis of PD independently; emerging attention is starting to be paid to the impact of pesticides on the microbiome in general. This review aims to integrate these separate perspectives together in the context of PD development, summarize the direct effects of PD-relevant pesticides on the CNS and the ENS and investigate their impact on the microbiome and microbiome-gut-brain axis, represented by both local and systemic effects of dysbiosis, including leaky gut with easier penetration of toxins through the intestinal barrier and multiple subsequent cascades mediated by bacterial metabolites. To remain practically and clinically oriented, an overview of possible future steps for translation follows, consisting of recommendations for future pesticide use and research.
METHODS
A search in Medline (Pubmed) was conducted using terms referring to Parkinson’s disease, pesticides and microbiome, as follows: (“Parkinson Disease” [Mesh] OR “Parkinson*” [tw]) AND (“Pesticides” [Mesh] OR “Agrochemicals” [Mesh] OR “Pesticide Residues” [Mesh] OR “Pesticide Synergists” [Mesh] OR “pesticide*” [tw]) AND (“Microbiota” [Mesh] OR “Brain-Gut Axis” [Mesh] OR “Microbiotas” [tw] OR “Microbial Community” [tw] OR “Community, Microbial” [tw] OR “Microbial Communities” [tw] OR “Microbial Community Composition” [tw] OR “Community Composition, Microbial” [tw] OR “Composition, Microbial Community” [tw] OR “Microbial Community Compositions” [tw] OR “Microbial Community Structure” [tw] OR “Community Structure, Microbial” [tw] OR “Microbial Community Structures” [tw] OR “Human Microbiome” [tw] OR “Human Microbiomes” [tw] OR “Microbiome, Human” [tw] OR “Microbiome” [tw] OR “Microbiomes” [tw] OR “gut-brain axis” [tw] OR “brain-gut axis” [tw]).
Additional relevant articles were detected in the citation lists of the papers identified by the literature search. All types of articles published in English up until December 2022 were evaluated for this narrative review.
DIRECT EFFECTS OF PESTICIDES ON THE NERVOUS SYSTEM
The recognition of a potential link between pesticides and PD dates back to the 1980s, when several individuals developed marked parkinsonism after intravenous illicit drug abuse. Exposure to 1-methyl-4-phenyl-1,2,3,6-tetrahydropyridine (MPTP), a substance structurally similar to the herbicide paraquat, led to selective damage of dopaminergic (DA) neurons in the substantia nigra (SN) [33]. Since then, several pesticides have been used in experimental and animal (mostly rodent) models of PD [34]: MPTP (selective death of DA neurons in the SN) [35], rotenone (inhibition of complex I in the respiratory chain, selective nigrostriatal DA degeneration, cytoplasmic inclusions similar to Lewy bodies, and motor symptoms) [36, 37], paraquat (dose-dependent degeneration of DA neurons in the SN) [38, 39], the combination of paraquat and maneb (degeneration of DA neurons in the SN, loss of striatal dopamine and reduced motor activity) [40], or dieldrin (increased α-syn expression and alterations in the DA system) [41]. Pesticide-induced PD animal models serve as a proof-of-principle concept that exogenous substances can produce selective degeneration of DA neurons in the SN, i.e., that environmental toxicants can have a direct role in development of PD [42]. Since the 1980s, multiple studies have unraveled the connection between pesticides and PD from both epidemiological [43, 44] and molecular perspectives; these were recently reviewed, e.g., by Yuan et al. [45], Vellingiri et al. [46], or Nabi et al. [47]. Apart from MPTP, the pesticides most implicated in PD include rotenone, paraquat, maneb, organochlorines, and pyrethroids. All of them are still widely used in agriculture or aquaculture as herbicides, fungicides, or insecticides [29]. Abundant application leaves residues or metabolites of pesticides in food, drinking or groundwater [48], indicating different possible effective entry routes for humans, though in most cases, it is the gut that is directly in contact with food contaminants [29]. However, in cases of occupational contact with pesticides, exposure via inhalation and ingestion has also been associated with a higher risk of late-life prodromal features of PD than dermal exposure only [49].
A clear distinction between toxin-induced parkinsonism and idiopathic PD may be complicated. Cases with acute-onset tremors, bradykinesia and rigidity following accidental or voluntary exposure, usually not progressing once the agent is stopped, with 10% to 20% even improving (described, e.g., in manganese, mercury, MPTP, organochlorines, organophosphates, rotenone, or paraquat exposure [50, 51]) clearly fall into the category of toxin-induced parkinsonism. However, it may not be possible to draw this dividing line so unequivocally in the case of epidemiological studies linking higher PD prevalence with certain geographic regions or occupations. A comparison to the genetic architecture of PD can be made—there is a genetic background continuum ranging from rare high penetrance gain or loss of function mutations (causing PD on their own, often with a clinical phenotype different from idiopathic PD [52]) to relatively common genetic risk variants that only mildly modify disease risk [53]. The same may be true for pesticides, depending on the intensity and duration of exposure. Cases of toxin-induced parkinsonism following acute high-dose accidental or intentional pesticide intoxication represent their direct causative effect [33, 54, 55], whereas increased PD prevalence among agricultural workers with chronic occupational exposure or in areas of chronic residential exposure [56] might represent their contribution to disease pathogenesis as a modifier of disease risk (although other factors are likely important, as not all equally exposed workers subsequently develop PD; pesticides might act as one piece of the mosaic in the multifactorial etiology of PD) [21, 57].
A recent quantitative epidemiological study aimed to identify PD-relevant pesticides; out of 288 specific pesticides available for PD risk evaluation in a pesticide-wide association study, long-term exposure to 53 of them was associated with PD, with 10 pesticides being directly toxic to DA neurons derived from PD patient-induced pluripotent stem cells. Co-exposures resulted in greater toxicity than any single pesticide, and trifluralin was found to be a driver of toxicity to DA neurons, leading to mitochondrial dysfunction [58]. These findings bring new pollutants into research attention and pave the way forward for understanding the effects of complex pesticidal exposure. However, as the evidence on their molecular mechanisms and impact on gut microbiota is currently missing, the focus of this review will be on pesticides with a long-recognized link to PD and their impact on disease pathogenesis through multiple pathways.
Central effects: toxicity on dopaminergic neurons in the substantia nigra
Pesticides associated with PD lead to α-syn pathology and degeneration of DA neurons through a number of molecular mechanisms, including oxidative stress, interference with dopamine transporters (DAT), mitochondrial abnormalities, stimulation of α-syn fibrillation and neuroinflammation [46]. The most relevant pesticides and their effects are mentioned in the following text.
Rotenone, a lipophilic isoflavonoid insecticide, exerts its detrimental effects on DA neurons mainly by inhibiting the function of mitochondrial complex I of the electron transport chain [59], resulting in ATP reduction and generation of excessive reactive oxygen species (ROS) and leading to cellular damage and death [60]. Aberrant mitochondrial dynamics (an increased ratio of mitochondrial fusion and fission) following long-term low concentration exposure was also described [61], as was externalization of cardiolipin to the mitochondrial surface and an increase in the expression of LC3, suggesting the involvement of autophagy [62]. Another mechanism is the inhibition of proteasome activity, leading to impaired protein degradation [63]; α-syn aggregation and phosphorylation, lessened tyrosine hydrolyze expression, decreased dopamine secretion [37, 64], changes in synaptic plasticity [65], upregulation of apoptotic pathways [66], microtubule deformation with subsequent disruption of vesicular transport [67], impaired iron homeostasis [68], alterations in the extracellular matrix [69] and several other mechanisms expand the list [70]. Although DA neurons are the most affected, neurochemical injury extends beyond DA regions –it causes damage to striatal serotonergic fibers, striatal projection neurons, cholinergic interneurons, the pedunculopontine tegmental nucleus and noradrenergic neurons in the locus coeruleus [71]. Non-neuronal mechanisms are also involved as well: oxidative stress and mitochondrial dysfunction involve astrocytes, microglia, vascular endothelial cells and pericytes, likely promoting damage to the DA neurons [72, 73]. Microglial activation actively participates in the rotenone-induced degeneration of DA neurons in mesencephalic neurons–glia cultures [74] and was even reported to precede it in a rat model [75].
Paraquat, a bipyridyl herbicide, acts primarily through excessive ROS production [76, 77]. Oxidative stress inhibits autophagy in astrocytes and interferes with their neuro-supportive effects [78], even causing them to become senescent and secrete soluble factors to promote the death of DA neurons [79]. Paraquat exposure leads to the accumulation of α-syn via inhibitory effects on proteasome, autophagy [80] and oxidative stress [81]. It also affects the transcription of neurogenesis-related genes in mice [82] and increases proinflammatory cytokine and interleukin-6 (IL-6) in human induced pluripotent stem cell-derived astrocytes in PD [79]. Interesting findings were detected when evaluating the effects of a combination of pesticides: maneb, a dithiocarbamate fungicide, has low toxicity on its own [83], but it was found to change the toxicokinetics of paraquat through inhibition of the mitochondrial complex III function in rat brain and mitochondrial dysfunction in primary mesencephalic neuron culture [84, 85]; thus their combination has a positive synergistic effect, resulting in neurotoxicity [86].
Glyphosate (Gly), an organophosphorus herbicide—the active compound in several formulations with surfactants, referred to as Gly-based herbicides (GBH), e.g. Roundup®—is the most widely used herbicide in agriculture, with increasing recognition of its effects on the nervous system [87]. Although previously considered to be a low risk compound for human health, as its main mechanism of action is the enzymatic inhibition of the shikimic acid pathway that is present in plants but not in mammals, it is now classified as probably being carcinogenic to humans (group 2A) [88] and directly neurotoxic for the CNS, inducing changes in the levels of monoaminergic neurotransmitters [89], generating an oxidative environment [90] or glutamatergic excitotoxicity [91]. Toxin-induced PD cases were described both after acute Gly intoxication (intentional ingestion-suicide attempt [54], accidental exposure [55]) and chronic occupational exposure [57]. Moreover, a 33% higher odds of premature death from PD in areas with chronic residential exposure to Gly in Washington state was reported [56]. Evidence from cell and animal models supports the vulnerability of dopamine-containing neurons to Gly: the dose- and time-dependent death of DA cells derived from pheochromocytoma cells via autophagy and apoptotic pathways was observed after Gly exposure [92], as well as exacerbation of MPTP neurotoxicity on DA neurons in the SN [93]. Other Gly effects in the SN were related to changes in neurotransmitter systems beyond dopamine, especially the reduction of opiate-related dynorphin peptides [91].
Organochlorines, chlorinated hydrocarbons including cyclodienes, hexachlorobenzene, polychlorinated biphenyls (PCB), dichlorodiphenyltrichloroethane and its metabolite, dichlorodiphenyl dichloroethylene, are persistent and bioaccumulative neurotoxicants that disrupt nerve fiber sodium/potassium currents in PD via energy metabolism abnormalities, chronic activation of sodium channels and interaction with γ-amino butyric acid (GABA) receptors [94]. PCB neurotoxicity is exerted by modified dopamine signaling, thyroid hormone signaling impairment, disturbance of calcium intracellular Ca2 + dynamics and oxidative stress [95]. Dieldrin exposure in mice led to higher oxidative damage, reduced DAT expression, enhanced α-syn expression [41], mitochondrial dysfunction [96], reduction of the proteasomal activity [97] and induction of histone acetylation [98]. Beyond that, in utero exposure to dieldrin results in genome-wide changes in DNA methylation and a long-term gene expression dysregulation, contributing to late-life neurodegeneration [99].
Pyrethroids, synthetic pesticides, including, e.g., deltamethrin, permethrin, or cypermethrin, cause neuronal damage in the hippocampus and striatum through increased production of ROS, resulting in necrosis or apoptosis [100]. The main neurotoxic mechanisms leading to nigrostriatal DA neurodegeneration include oxidative stress, inflammation, apoptotic neuronal cell loss and mitochondrial dysfunction; the main neurodegeneration targets are ion channels (activation of sodium channels, inhibition of GABA receptor-gated chloride channels). Permethrin was found to increase α-syn and decrease striatal dopamine levels [101]. Exposure to 3-phenoxybenzoic acid (3-PBA), a prominent metabolite of most pyrethroids, could mimic the pathological and pathogenetic features of PD, acting as a key pathogenic compound in pyrethroid-related pathological effects and a possible dopamine neurotoxin [102]. However, epidemiological evidence for a direct role of pyrethroids in PD development is rather limited [60, 103].
Taken together, there is very strong evidence from rodent models linking pesticides to dopaminergic toxicity, particularly for rotenone and paraquat.
Peripheral effects: toxicity on the gut and enteric nervous system
Gut dysfunction and the gut-brain axis are of particular interest due to their possible involvement in the pathogenesis of PD [104]. In terms of the discovered nigro-vagal pathway [105], ingested environmental toxicants could enter the CNS through vagal connections from the gut and induce or accelerate PD progression [104]. In fact, beyond pure DA neuronal damage in the SN, peripheral toxicity, and alterations of the ENS/PNS were described after exposure to several pesticides, as outlined in the following text.
In a rotenone rat model of PD, α-syn aggregates in the ENS and gastrointestinal (GI) dysfunction were detected [106], as well as a reduced intestinal transit associated with α-syn aggregates and intestinal inflammation in a PD mouse model [107]. Rotenone exposure led to a substantial decrease in the number of myenteric neurons and a significant delay in total GI transit time [108], gastric emptying [36], and stool frequency [109, 110]. Also, it has been proposed that rotenone promotes the release of α-syn from mouse myenteric neurons and its uptake by presynaptic vagal fibers, which act as its conduit to the brainstem; α-syn subsequently decreases the number of preganglionic parasympathetic cholinergic neurons in the dorsal nucleus of the vagus nerve (DMV) [111]. This progression of α-syn ascent based on transneuronal and retrograde axonal transport was stopped by vagotomy, which suggests a sufficient effect of disconnecting the gut–brain axis to halt the process in animal models. Apart from myenteric neurons, enteric glial cells (EGCs, commonly referred to as the astrocytes of the gut) also play a significant role in the PD-related pathology of the gut [112], reactive enteric gliosis and its proinflammatory phenotype [113]. In a rat model, both rotenone and tebufenpyrad (Tebu, a pyrazole greenhouse acaricide, another mitochondrial complex I inhibitor [114]) led to impaired mitochondrial fission and fusion, increased ROS production and caused mitochondrial dysfunction, as well as induced proinflammatory signaling and autophagic dysfunction in EGCs, ultimately leading to ENS dysfunction [115].
In paraquat-treated rats, misfolded α-syn was found in both myenteric and DMV neurons [105, 116], although without affecting the number of gastric myenteric cholinergic neurons. A substantial attenuation of the gastric motility response to stimulation of the nigro-vagal pathway was described [105]; however, rather than the expected inhibitory-only effects on the stomach, a maladaptive increase in the excitability of DMV neurons [117] and an altered response to dopamine, which results in a biphasic (stimulatory followed by inhibitory) gastric response, seems to be the explanation [118]. In a paraquat-induced PD mouse model, several mechanisms involved in PD gut dysfunction were replicated: impaired intestinal barrier (decreased expression of ZO-1 (zonula occludens 1) and occludin), inflammation (increased expression of CD11b, iNOS, HMGB1, and IL-1β) and the presence of α-syn pathology [119].
In models studying glyphosate exposure, it was also proposed that neurotoxic Gly effects may be related to a disruption of the gut-brain axis [120], although mostly via dysbiosis; evidence for impairment of the ENS itself (e.g., alterations in the neurochemical code of enteric neurons in the porcine duodenum) is very scarce and unrelated to PD [121].
The extent of current knowledge about the impact of various pesticides on the ENS/PNS in PD is still rather limited compared to the level of evidence of their central neurotoxicity to DA neurons in the SN. However, this research area may be of particular interest in the context of increasing knowledge on the role of the microbiome-gut-brain axis in PD development, as discussed in the next section.
PESTICIDES AND THE MICROBIOME-GUT-BRAIN AXIS
The microbiome-gut-brain axis in PD
The prodromal phase of PD
To elucidate potential options about how pesticides might influence PD pathology and progression, it is important to outline how PD pathology progresses throughout the nervous system before a diagnosis can be made based on the onset of cardinal motor symptoms. It is now accepted that PD has a long prodromal phase in which pathology spreads throughout the peripheral and central nervous system before a wide-range pathology in the SN results in the onset of cardinal motor symptoms [122]. The “dual hit hypothesis”, proposed in 2007, suggested a nasal route (with olfactory bulb involvement and anterograde progression to the temporal lobe) versus a gastrointestinal route of entry (with propagation of pathology via the vagus nerve) [123]. In fact, both hyposmia and gut dysfunction are common non-motor symptoms not only in manifest PD, but also preceding the onset of motor symptoms by years [124]. The gut has become particularly interesting as an early disease indicator, with constipation being the earliest non-motor symptom documented (up to 20 years prior to onset of motor symptoms, even before hyposmia) [125]. Indeed, seeding the duodenum with α-syn preformed fibrils in a mouse model led to disruption of ENS connectivity and time-dependent GI dysfunction [126]. In humans, α-syn deposits in GI tissues have been observed up to 20 years prior to a PD diagnosis [127], although we still lack full understanding of the diversity of α-syn misfolded forms, which does not allow its use as a reliable PD biomarker yet, despite significant efforts to develop a standardized diagnostic tool [128, 129]. Thus, at least in a subtype of PD patients the gastrointestinal tract (GIT) may be the starting point where neuroinflammation and neurodegeneration leading to PD pathology might be triggered. Environmental pathogens might then gain access through the GIT, disrupting the ENS and leading to α-syn pathology, which could reach the brain in a prion-like manner via the vagus nerve [130, 131].
Two basic different PD subtypes have been proposed in regards to the trajectory of disease progression: the so-called brain-first and body-first PD [132]. In the proposed brain-first PD the initial α-syn misfolding happens in the CNS with subsequent descendent spreading [133]. In contrast, the body-first subtype is characterized by initiation of α-syn misfolding in the gut (ENS/PNS) and ascendent α-syn pathology through the vagus nerve to the brainstem and higher [122], and changes in the microbiome-gut-brain axis induced by pesticide exposure are likely more relevant for this phenotype, as discussed in the following text.
Enteral dysbiosis in PD
Gut health is a precondition for brain health; alterations of the gut microbiota composition are observed to co-occur with many neurological diseases [134]. The gut microbiome, composed of thousands of diverse microbial species, is involved in multiple complex interactions with the human body, including metabolism (synthesis and degradation of vitamins, amino acids, lipids, bile acids (BA) and neurotransmitters; fermentation of indigestible food substances, xenobiotic metabolism), immune function (stimulation and/or regulation of immune responses) or structural function (regulation of epithelial cell growth and the intestinal barrier) [135]. Host-microbiome crosstalk has come into focus as the key mediator of neurological health; there is evidence that gut microbiota regulate the development of the blood-brain-barrier (BBB) and influence the function of microglia and astrocytes [136]. Recent reviews have summarized the role of gut microbiota in risk of developing neurodegenerative diseases [137, 138] and PD in particular [139, 140], even focusing on the prodromal period [141]. Taken together, a number of studies have detected alterations in gut microbiota composition in PD patients, with subsequent changes in their products and metabolites. In the summary of Tan et al., at least 42 families, 102 genera and 44 species of bacteria were reported to be differentially abundant in PD patients compared to controls [139]. From a functional point of view, this dysbiosis might result in a pro-inflammatory status which could be linked to the recurrent GI symptoms affecting PD patients.
Local and systemic effects of dysbiosis in PD: “leaky gut”, inflammation, and α-syn pathology
Intestinal integrity is crucial for proper gut function. The semipermeable gut barrier limits the transport of harmful substances (including environmental pollutants), regulates absorption of nutrients and allows immune sensing, whereas a “leaky” gut, a condition of compromised intestinal barrier seen in PD, results in an increased flux of both microbes (including bacteria) and molecules (including toxins) across the intestinal epithelium. Various studies support the concept of intestinal hyperpermeability and inflammation in PD, including among others increased fecal markers of intestinal inflammation [142, 143] and higher pro-inflammatory gene profiles associated with intestinal barrier disruption [144]. Dysbiotic gut microbiota expressing more lipopolysaccharides (LPS) can overstimulate the toll-like receptors (TLR) expressed on epithelial, immune and enteric glial cells, which together with higher intestinal barrier permeability further provokes local and systemic inflammation and enteric neuroglial activation, triggering the development of α-syn pathology and ultimately not only gut, but also brain inflammation [113, 145–150].
It has been suggested that α-syn participates in innate and adaptive immunity [151, 152], and inflammation is connected to an important feedback loop that promotes its spread –an inflammatory environment enhances α-syn expression, misfolding and aggregation [148, 153–158], which in turn induces local proinflammatory immune responses [126, 147, 152, 159, 160]. Gut microbes, besides contributing to inflammation, can play an additional mechanistic role in the process, as microbial curli (extracellular amyloid proteins produced and expressed abundantly by certain bacteria, such as Escherichia coli) have been suggested to template α-syn aggregation through cross-seeding mechanism in the gut [161, 162].
Tan et al. summarized the whole process as a vicious cycle where dysbiosis, hyperpermeability, inflammation, and α-syn aggregation drive and perpetuate one another [139]. Notably, as gut inflammation and expression of α-syn might be common events during life, other additional contributing factors are proposed as having importance for driving PD pathogenesis in vulnerable persons, including environmental influences [163, 164].
According to the current understanding, there is a process that begins locally—leaky gut and dysbiosis-induced gut inflammation (detected in both colonic biopsies and fecal samples from PD patients [142–144, 148–150]) which then continues to drive disease pathogenesis through several systemic mechanisms, such as increased cytokine production, BBB disruption, migration of inflammatory cells into the brain [160] and microglial activation, finally culminating in neuronal dysfunction and/or loss [165].
Pesticides affect the microbiome: consequences of pesticide-induced dysbiosis
The impact of environmental pollutants (and pesticides in particular) on gut health has started to gain recognition in recent years [30]. There is sufficient evidence that pesticides can impair gut barrier function by destroying intestinal mucosa and affecting the crosstalk between mucus and gut bacteria [166, 167]. They alter the overall composition of gut microbiota and affect their metabolites, leading to pathological changes by acting on receptor sites of different tissues and organs [168]. It is time to seriously consider the gut microbiota as an unintended recipient of pesticidal pollution and to focus on the long-term effects of chronic exposure on microbial diversity and subsequent influence on the host [29]. Table 1 shows a summary of the shifts in gut microbial composition following exposure to several PD-relevant pesticides in various experimental animal models, which can also be found in the following section.
Table 1
Shifts in the gut microbial composition following exposure to PD-relevant pesticides in various experimental models
Pesticide | Model | Administration | Shifts in gut microbiota composition | Reference |
Rotenone | Mouse | Oral gavage | ↓ Firmicutes-to-Bacteroidetes (F/B) ratio, ↑ Rikenellaceae and Allobaculum; ↓ Bifidobacterium in both the caecal mucosa-associated and luminal microbiota community structure | [169] |
Oral | overall ↓ in bacterial diversity, significant microbial shift at the main bacterial phyla, resulting in ↑ F/B ratio | [170] | ||
Gavage | ↑ genera Lactobacillus, Bifidobacterium, Akkermansia, and Bacteroides species, ↓ Lachnospiraceae, Ruminococcaceae, Turicibacter, Faecalibaculum, and Clostridium species | [171] | ||
Rat | Intraperitoneal | ↑ Bifidobacterium | [173] | |
Paraquat | Mouse | Intraperitoneal | relative abundance significantly different in 10 dominant bacterial groups, ↓ Lachnospiraceae, Ruminococcaceae and Rikenellaceae, ↑ Bacteroides after 4 weeks; ↓relative abundance of Prevotellaceae already after 2 weeks. | [182] |
↓ gut microbiota diversity of adult mice males | [183] | |||
Glyphosate | Mouse | Oral gavage | ↓ Corynebacterium, Firmicutes, Bacteroides, and Lactobacillus | [188] |
Rat | Oral, in tap water | ↑Bacteroidetes over Lactobacillus | [189] | |
Rat pups | Maternal exposure in drinking water | ↑ Bacteroidetes (Prevotella), ↓Firmicutes (Lactobacillus) | [190] | |
Honey bee | Feeding | ↓ of the bacterial community and weakening in beneficial bacterial composition | [191] | |
Poultry microbiota | In vitro exposition | beneficial intestinal bacteria –Enterococcus faecalis, E. faecium, Bacillus badius and B. cereus sensitive to Gly, pathogenic bacteria –E. coli, Salmonella enteritidis, S. typhimurium, S. galliarum, Clostridia species resistant to Gly | [192] | |
Cow | Added to the rumen | ↓ Entodinium, Epidinium, Ophryoscolex and Dasytricha species | [193] | |
no changes | [194] | |||
Horse, cattle | Oral | ↓ Enterococcus species, which altered its inhibitory role against Clostridium botulinum | [195] | |
MPTP | Mouse | Subcutaneous injection | ↓ abundance and diversity, ↑ Ruminococcus, Parabacteroides and Parasutterella genera, ↓ Coriobacteriaceae, Flavonifractor, Lachnospiraceae, Lactobacillaceae and Rikenellaceae | [221] |
Intraperitoneal injection | ↓ Lachnospiraceae, Clostridiales and Proteobacteria, ↑ Erysipelotrichaceae, Prevotellaceae and Erysipelotrichales | [222] | ||
↓ Bacteroidetes phylum, Lactobacillales order and Prevotellaceae family | [223] | |||
↓ Firmicutes (order Clostridiales), ↑Proteobacteria (order Turicibacterales and Enterobacteriales) | [224] | |||
Chlorpyrifos | Rat | Oral | ↓ Firmicutes and Bacteroidetes | [226] |
SHIME | In vitro exposition | ↑ Enterococcus and Bacteroides, ↓ Bifidobacterium and Lactobacillus | [228] | |
Permethrin | Rat | Gavage | ↓ Firmicutes and Bacteroidetes | [227] |
Rotenone
In a rotenone-induced mouse model of PD, a clear shift towards putative pro-inflammatory dysbiosis was observed in both the cecal mucosa-associated and luminal microbiota community structure. Changes in β-diversity were represented by a decreased Firmicutes-to-Bacteroidetes (F/B) ratio, increased relative abundance of putative pro-inflammatory bacteria, such as Rikenellaceae and Allobaculum, and decreased relative abundance of putative anti-inflammatory bacteria, such as Bifidobacterium. Dysbiotic microbiota correlated with PD-like functional and pathological changes in both the gut and the brain, as seen in the association of above-mentioned bacterial families with intestinal epithelial barrier integrity (assessed by ZO-1 expression), inflammation (assessed by the number of CD3 + T-cells in the colon), α-syn accumulation in the colonic plexi and the number of DA neurons in the SN. Moreover, rotenone induced a significant reduction of several metabolic pathways in the gut microbiota: biodegradation and metabolism of xenobiotics, as well as metabolism of cofactors, vitamins, carbohydrates, amino- and fatty acids. Another study in a mouse model observed rotenone-induced GI and motor dysfunctions that correlated with changes in fecal microbiota composition; dysbiosis was characterized by an overall decrease in bacterial diversity and a significant microbial shift in the main bacterial phyla, resulting in an increased F/B ratio [170], the last being in contrast with the previous study. The differences were explained by possible effects of different rotenone oral dose concentrations, different GI sites and different sequencing techniques [169]. Another study found an increase in the relative abundance of species of the genera Lactobacillus, Bifidobacterium, Akkermansia, and Bacteroides and a decrease in species of Lachnospiraceae, Ruminococcaceae, Turicibacter, Faecalibaculum, and Clostridium in rotenone-treated mice [171]. Several of these shifts (increased Lactobacillaceae and Bacteroides and decreased Lachnospiraceae) were consistent with findings from human PD studies, where they were associated with worsening of clinical symptoms, such as gait disturbances or postural instability [172].
In a rat model, intraperitoneal rotenone administration led to alterations of small intestinal and colonic microbiome composition (although most of them failed to reach statistical significance, with the exception of increased Bifidobacterium in the colon) and reproduced clinical symptoms of gastroparesis before nigrostriatal pathology was evident [173]. Several microbial shifts were in line with changes reported in human PD patients: increased Lactobacillus and Bifidobacterium [174–177] and increased Clostridiaceae and reduced Lachnospiraceae and Prevotellaceae [175, 178, 179]. Decreases in the Prevotellaceae family and increases in the Lactobacillaceae and Bifidobacteriaceae families are of particular interest, as these changes have been associated with reduced serum ghrelin [177, 180], a gut hormone that reportedly regulates nigrostriatal dopamine function and thus may be able to attenuate neurodegeneration in PD [181]. Thus, animal rotenone PD models seem to replicate human PD-related dysbiosis, with subsequent inflammatory changes in the gut and an influence on further metabolic pathways.
Paraquat
Intraperitoneal paraquat administration in a mouse model of PD led to alterations in gut microbiome diversity: the relative abundance was significantly different in 10 dominant bacterial groups, with Lachnospiraceae, Ruminococcaceae, and Rikenellaceae being the lowest and Bacteroides the highest after four weeks, while the relative abundance of Prevotellaceae was significantly lower already after two weeks. Besides gut microbiota disruption, intestinal epithelial barrier damage (seen as decreased expression levels of colonic tight junction proteins) and inflammatory responses (seen as increased expression levels of inflammatory markers) were suggested to be the main causes of gut dysfunction, and pathological aggregation of gut-derived α-syn and disturbance of short-chain fatty acids (SCFA) in the gut might be the key components in communication of the gut-brain axis, leading to pathological changes in the CNS after paraquat exposure [182]. Interesting results were observed in another study: postnatal paraquat intraperitoneal injection in mice increased the body weight and reduced the gut microbiota diversity of adult mice males, with gene function prediction analysis suggesting that the two observed outcomes were highly correlated [183]. Although this was not a mouse model specific for PD, it demonstrates that early-life paraquat exposure can disturb the gut microbiota and result in long-term effects in a sex-specific manner, and given that obesity, metabolic syndrome and type 2 diabetes are risk factors for PD [184] and that PD more frequently affects men, these findings might also be relevant.
Glyphosate
Mode of action, alterations in the gut microbiota composition: Several effects of glyphosate (Gly) are proposed to be mediated by inducing alterations in gut microbiome composition. Gly (herbicide) acts as a competitive inhibitor of 5-enolpyruvylshikimate-3-phosphate (EPSP) synthase, an enzyme involved in the shikimate pathway responsible for the synthesis of aromatic amino acids (phenylalanine –Phe, tyrosine –Tyr and tryptophan –Trp) in plants, essential for cellular survival [185]. Gly was previously considered to be a safe compound for humans, as EPSP synthase is absent in animals and humans, who depend upon ingested food and gut microbes to provide these essential nutrients [120]. However, the shikimate pathway is essential to the metabolism of some species in the human gut microbiome. A conservative estimate is that 54% of species in the core human gut microbiome are sensitive to Gly [186]. Thus, Gly exposure leads to enzymatic inhibition in bacteria and subsequent gut dysbiosis, which causes behavioral impairments [187].
A number of studies detected various gut microbiome imbalances in animal models, although not specifically related to PD. In mice, a significant alteration in gut microbiota composition, abundance and phylogenetic diversity was observed for Corynebacterium, Firmicutes, Bacteroides and Lactobacillus, all reduced by Gly exposure; furthermore, increased anxiety and depression-like behavior occurred after subchronic and chronic exposure to GBHs herbicides [188]. In a rat model, dysbiosis induced by Gly was characterized by an increase in Bacteroidetes over Lactobacillus, with a sex-specific effect, principally affecting female rats [189]. Similar results were also seen in rat pups after maternal exposure to GBHs in drinking water. The relative abundance of Bacteroidetes (Prevotella) was increased, and Firmicutes (Lactobacillus) was reduced [190]. A reduction of the bacterial community and a weakening of beneficial bacterial composition was observed in honey bees, which makes them more susceptible to infection by Serratia, an opportunistic pathogen causing mortality [191]. In poultry microbiota, a variable effect on different bacterial strains was documented: beneficial intestinal bacteria, such as Enterococcus faecalis, E. faecium, Bacillus badius and B. cereus, were found to be sensitive to Gly, whereas the Clostridia species and pathogenic bacteria, such as E.coli, Salmonella enteritidis, S. typhimurium or S. galliarum, showed marked resistance to Gly [192]. Varying results were found in cow rumen: a reduction in Entodinium, Epidinium, Ophryoscolex, and Dasytricha species [193] compared to no changes in another study [194]. In horse and cattle, Gly caused low levels of Enterococcus species, which altered its inhibitory role against Clostridium botulinum [195]. A predisposition to the development of Clostridium tertium bacteraemia in humans with an effect on the intestinal mucosa was indicated after a voluntary ingestion of Gly (a suicide attempt by a human female) [196]. Consequently, it has been suggested that Gly can cause dysbiosis [120]. However, relevance for the human gut microbiome remains questionable. Most gut bacteria do not possess a complete shikimate pathway; this pathway is mostly transcriptionally inactive, suggesting that gut bacteria are mostly aromatic amino acid auxotrophs (in conditions of adequate dietary quantity) and are thus relatively resistant to potential growth inhibition induced by Gly. Moreover, one-fifth of E. coli EPSPS enzyme homologues have been found to be resistant to Gly, and antimicrobial effects were mostly found at high doses unlikely to occur in real-world exposure scenarios [197].
Alterations in neurotransmitter metabolism: There are several mechanisms that are unique to Gly among all emergent herbicides. Inhibition of the shikimate pathway not only has deleterious effects on gut microbiota metabolism and survival itself, but also leads to reduced synthesis of essential aromatic amino acids humans depend on. Apart from Trp, Tyr and Phe, there are other biologically active molecules that need shikimate pathway metabolites as precursors (serotonin, melatonin, melanin, epinephrine, dopamine, thyroid hormone, folate, coenzyme Q10, vitamin K, vitamin E); methionine and glycine are also negatively impacted by Gly [198].
Gut bacteria interact with the host nervous system through several neurotransmitters or their metabolic precursors [199], including GABA and Trp, the latter of which is a central precursor of serotonin (5-hydroxytryptamine, 5-HT), a neurotransmitter involved in many different physiological processes and psychoaffective functions [200]. The gut microbiota has already been repeatedly shown to participate in modulation of 5-HT turnover [201]. Trp is generated by the gut microbiota and is converted to 5-HT after crossing the BBB [202]. Returning to the shikimate pathway again, Gly acts as an inhibitor of EPSP synthase, the rate-limiting step in the synthesis of aromatic amino acids, including Trp [198]. Indeed, Gly effects in altering gut microbiota composition with subsequent neurobehavioral consequences were implicated in several conditions, such as depression and anxiety [188] or autism spectrum disorders [203], reviewed in [120], even in case of maternal exposure with outcomes in the offspring, suggesting that microbiota could regulate neurobehavior by modulating the development of CNS serotonergic neurotransmission [188]. Given that PD is a multi-transmitter neurodegenerative disorder with a significant role of serotonergic signaling in many non-motor symptoms, mainly neuropsychiatric [204, 205], this mode of action of Gly might also be of potential relevance in PD.
Manganese metabolism interference: Manganese (Mn) seems to be another piece of the mosaic regarding complex PD risk from environmental exposure. Manganism, a condition closely resembling PD (with similar clinical manifestation, although some differences in underlying neuropathology—the absence of Lewy bodies or different distribution of neuronal loss—in the locus coeruleus, globus pallidus and pars reticulata of the SN [206, 207]) develops after chronic occupational Mn exposure [208, 209], and there is an increase in the incidence of PD in urban areas with a higher industrial release of Mn [210].
Gly acts as a Mn chelator, thus interfering with its metabolism. Severe Mn depletion can be another limiting step for the shikimate pathway in plants and bacteria, as Mn is a catalyst for EPSP synthase [211]. Disruption of Mn homeostasis can selectively affect Lactobacillus, which utilizes Mn as a protective mechanism against oxidative damage; thus, its requirements for Mn are much higher compared to other species [212], which can lead to its reduced number in the gut. This may be related to another neurotransmitter-linked microbiome-gut-brain mechanism. Several members of the Lactobacillus family can produce GABA, the main CNS inhibitory neurotransmitter implicated in several conditions such as anxiety or depression, possibly relevant also in PD, and alterations in GABA neurotransmission in PD have gained recognition in many disease-related symptoms [213]. Probiotic treatment with L. rhamnosus restoring central GABAergic function could be of interest as a potential novel therapeutic approach to (PD-related) depression [214].
It might seem contradictory that Gly exposure could be linked to PD pathogenesis involving Mn, given that glyphosate acts as Mn chelator. However, Gly impairs Mn metabolism by several mechanisms, and some of them could be related to dysbiosis. In the review of Samsel and Seneff it was proposed that, paradoxically, both Mn deficiency and Mn toxicity attributable to Gly can occur simultaneously [211] and that this disruption of Mn homeostasis leads to extreme sensitivity to its availability [215]. In liver, regulation of Mn levels in general vascular circulation is ensured by incorporation of its excess amount into BA, thus providing the gut bacteria repeated access to Mn, a process that is blocked by Gly due to its disruption of cytochrome P450 (CYP) enzymes, which are crucial for BA production [198, 216]. Mn accumulation in the liver can result in its transport via the vagus nerve to brainstem nuclei, causing neuronal damage leading to parkinsonism; this pathway could be related to Mn toxicity in the brainstem nuclei in the conditions of Mn abundance and the simultaneous presence of Gly [211].
Thus, on one hand, decreased serum levels of Mn, impairment of several Mn-dependent enzymes and its deficiency in brain areas apart from the brainstem are caused by Gly-mediated direct chelation of Mn as well as reduced bioavailability of Mn to the gut bacteria due to its failure to be incorporated into BA and its accumulation in the liver. On the other hand, the brainstem suffers from excess Mn levels. In the brain, Mn is preferentially localized in astrocytes; its overexposure can lead to Mn-induced excitotoxic neuronal injury by dysregulating astrocytic cycling of glutamine (Gln) and glutamate (Glu). In addition, reactive astrocytes are important mediators of Mn-induced neuronal damage by triggering neuroinflammatory responses [217]. Moreover, high concentrations of neuromelanin are found in both the SN and the locus coeruleus, and neuromelanin was also proposed to have a protective function via accumulating and retaining various amines and metallic cations, especially Mn [218]. As neuromelanin is related to dopamine (derived from Tyr) in the SN and to noradrenaline (derived from Trp) in the locus coeruleus, both dependent on shikimate pathway products, it was hypothesized that in case of Gly exposure and shikimate pathway inhibition in gut bacteria, neuromelanin is likely to be deficient, which would result in a reduced ability to temporarily store excess Mn in the brainstem nuclei until it can be disposed of [211].
There was a critical response stating that the relationship between Gly and Mn may be exaggerated in several aspects based on epidemiological studies rather than experimental evidence, indicating correlation, not necessarily causation. Thus, a stronger level of evidence is needed for the future final call for action (a potential global ban on Gly) [219].
Inflammation: Alterations in immune system function and promotion of inflammation can be another consequence of changes in gut microbiota. The effects of Gly and GBHs on immune function in different animals and isolated immune cells were recently reviewed, and it was concluded that many of them are mediated via changes in gut microbiota, with multiple proinflammatory effects (especially in fish) [220].
Other pesticides and toxins –MPTP, chlorpyrifos, permethrin
Several studies have also shown gut microbiota alterations in the MPTP-induced PD mouse model, such as decreased abundance and diversity with an increase of Ruminococcus, Parabacteroides, and Parasutterella genera and a decrease of Coriobacteriaceae, Flavonifractor, Lachnospiraceae, Lactobacillaceae, and Rikenellaceae (overall dysbiosis was associated with disturbance of the DA, kynurenine, and 5-HT metabolic pathways) [221], a change in the abundance of Lachnospiraceae, Clostridiales, and Proteobacteria (decreased) and Erysipelotrichaceae, Prevotellaceae, and Erysipelotrichales (increased) [222], a decrease in the Bacteroidetes phylum, the Lactobacillales order, and the Prevotellaceae family [223] or a decrease in the phylum Firmicutes (order Clostridiales) together with an increase in the phylum Proteobacteria (order Turicibacterales and Enterobacteriales) [224], with many findings showing consistency with observations in PD humans (increased abundance of Proteobacteria [179] and Enterobacteriales [225]).
An alteration of the gut microbiota composition (a decreased abundance of Firmicutes and Bacteroidetes) was also described in animals exposed to other pesticides, such as chlorpyrifos [226] and permethrin [227]. The first study was also validated in an in vitro SHIME model mimicking the human intestinal environment and demonstrating an increase in the numbers of Enterococcus and Bacteroides as well as a decrease in the numbers of Bifidobacterium and Lactobacillus after chronic exposure to low levels of chlorpyrifos [228].
Additional epidemiological data from a Norwegian cohort study revealed a potential influence on infant gut microbial function during a critical developmental window by uptake of low level pesticides from breast milk of a mother exposed to toxicants [229]. In detail, several Lactobacillus species were lower in abundance in samples from infants with relatively “high” vs. “low” toxicant exposure. As already discussed, Lactobacillus in general appears to be a genus that is highly prone to the effects of pesticides and environmental toxins.
Taken together, pesticide-induced dysbiosis and the shift to putative pro-inflammatory gut microbiota composition in general can (further) disrupt epithelial integrity, leading to gut leakiness, innate immune activation and possibly systemic inflammation [230–232]; moreover, there are some mechanisms unique to particular pesticides that lead to alterations of certain metabolic pathways or interference with neurotransmission.
Oral microbiome and the link to pesticides exposure
The oral microbiome has been studied far less compared to its gut counterpart, yet it might represent another overlooked missing piece in the overall picture of how environmental exposures impact human microbial communities. In a study of farmworkers occupationally exposed to organophosphates (insecticide azinphos-methyl), large-scale significant alterations of the oral buccal microbiota composition were detected, with extinctions of whole taxa suggested in some individuals (especially the Streptococcus genus) and long-lasting effects in repeated sampling (spring/summer –winter) [233]. Whether these changes could also be relevant in PD and might represent one of the early changes in microbiota composition occurring along the GI tract in response to ingested pollutants is not clear yet and requires further study.
The nasal microbiome, for instance, was shown to be relatively uninformative as a source of biomarkers for PD/iRBD (idiopathic REM Sleep Behavior Disorder, most relevant prodromal marker of PD) [234].
Microbiota mediate the effects of pesticides
Pesticide-induced dysbiosis represents only half of the reality; on the other side of the coin are multiple ways that the gut microbiota enable and modify the effects of pesticides by influencing their availability and metabolism, including many systemic pathways mediated by microbial metabolites and products.
Microbiota: presence required for pesticide toxicity, composition mediates toxin effects
It is widely agreed that consistent changes appear in the gut microbiota composition related to PD. However, this poses a chicken or egg question—does the underlying α-syn pathology affecting the ENS cause PD-related constipation, which in turn leads to dysbiosis, or do the alterations in gut microbiome due to multiple environmental factors lead to inflammation, α-syn accumulation, changes in the ENS, subsequent constipation and ascending PD pathology? Some hints come from animal models. In an α-syn overexpressing mouse model, germ-free or antibiotic (ATB)-treated animals were protected against neuroinflammation and motor dysfunction, whereas transplantation of stool from PD patients to these germ-free α-syn transgenic mice enhanced PD-like motor deficits and CNS pathologies significantly compared to microbiota transplants from healthy human donors. This suggests that gut bacteria are involved in PD pathogenesis to a high degree, and that dysbiosis alone can trigger PD pathology in a genetically susceptible host [163].
In the past, caution was raised due to the surprisingly high success rate of microbiome transfer experiments in germ-free animals in other disease models besides PD [235]. Whether the role of the gut microbiome was not being overstated and causality inferred in a false positive way was questioned. However, more studies followed in line with the first findings, and they indicated that microbiota act as an interface between the host and environmental exposures, and their presence and composition can alter the effects of pesticides both for better and for worse. Similar to the findings of Sampson et al. proving that fecal transplants from human PD subjects enhanced PD pathology in α-syn overexpressing transgenic mice (without any other external toxin exposure) [163], Sun et al. demonstrated that fecal transplants from MPTP-induced PD mice caused impaired motor function and decreased striatal dopamine and serotonin levels in control mice. Moreover, FMT from control mice to MPTP-induced PD mice improved their gut microbial dysbiosis and exerted neuroprotective effects, as evidenced in reduced motor impairment, elevated DA levels, reduced loss of DA neurons and restoration of 5-HT synthesis. The mechanisms underlying these beneficial effects were decreased activation of brain microglia and astrocytes and lowered fecal concentrations of SCFA, all mediated by inhibition of the TLR4/TBK1/NF-κB/TNF-α signaling pathway, thus suppressing neuroinflammation and gut inflammation [224]. Another study in a PD mouse model showed that ATB-induced microbiome depletion was protective against MPTP-induced DA neurotoxicity in the brain via the gut-brain axis [236]. Rotenone models yielded results consistent with previous studies—chronic rotenone treatment changed the gut microbiota composition significantly and caused an increase in intestinal permeability in conventionally raised mice, whereas no disruption of intestinal permeability was seen in germ-free mice, which again highlights the role of gut microbiota in regulating barrier dysfunction and motor deficits in PD [171].
These findings support the role of the microbiome in PD pathogenesis not only in transgenic animals with α-syn overexpression, but also in the context of exposure to environmental toxins. The gut microbiota is not only required for its neurotoxicity but also for mediating the effects of pesticides on intestinal permeability and the development of motor symptoms [171].
Mechanisms involved in microbiome-pesticide-host interactions
Huang postulated a hypothesis of “gut microenvironment baseline drift”, stating that the gut microbiota is a temporarily combined cluster of species sharing the same environmental stresses for a short period, which would change quickly under the influence of different environmental factors [237]. On the one hand, gut physiological properties and microbiome composition may be altered by hazardous environmental factors, such as pesticides [29], as shown in the previous section (4.2). On the other hand, the microbiome can adapt to environmental insults for improved survival by altering its composition, increasing the number of mutations in its genome or generating metabolites and products inducing various cascades that can be either beneficial (SCFA) or detrimental for the host (proinflammatory cytokines including IL-1b) [237].
Leaky gut: enabling/increasing pesticide toxicity: Besides the outcomes of dysbiosis, such as leaky gut, intestinal inflammation and α-syn misfolding, contributing to PD on their own [125, 145], there is one more important consequence to mention: intestinal hyperpermeability is likely to expose the intestinal neural plexi to toxins, including pesticides [238]. Two major barriers should prevent neurotoxins from entering the CNS: the BBB and the intestinal wall in the absence of gut dysbiosis. Once dysbiosis is present, it increases the permeability of the intestine (leading to increased entry of neurotoxins) and the BBB as well, enabling deleterious effects of neurotoxins, including pesticides, on the CNS, leading to neurodegeneration [239]. Pesticides therefore create a mechanistic self-perpetuating vicious cycle in which they first cause gut dysbiosis leading to inflammation and impaired intestinal barrier, which in turn allows increased exposure to pesticides (and other environmental pollutants and putative pathogens as well), further enabling and increasing their toxicity.
Xenobiotic metabolism: Gut microbiota play a crucial role in the degradation and metabolism of xenobiotics, i.e. chemicals to which an organism is exposed that are not naturally present or produced in that organism [240]. The gut microbiome can change the pharmacokinetics of xenobiotics (including pesticides) in multiple steps, affecting their bioactivity and toxicity, e.g., by inducing direct chemical modification leading to increased metabolism or bioactivation and reversing the modifications imparted by host detoxification pathways or by regulating host gene expression [241, 242]. In PD, increased metabolic activity of the microbial pathways involved in the degradation of xenobiotics, including herbicides (namely atrazine), was found in stool samples compared to controls [175]. Several Proteobacteria in the human gut microbiome have been found to degrade glyphosate using the carbon–phosphorus lyase pathway [197].
Metabolic/endocrine pathways: It was discovered that pesticides can induce obesity and endocrine disruption, all mediated by the gut microbiome and its metabolites [243]. There are many converging pathways involved, including insulin resistance, obesity, lipid metabolism, atherosclerosis, appetite changes and others [28, 237]. Although studies on the mechanisms by which pesticides can affect human metabolism through influencing the gut microbiota were not directly focusing on PD, given that obesity, metabolic syndrome and type 2 diabetes are risk factors for PD [184] and insulin resistance is one of the hallmarks of PD [244], these factors may emerge as important indirect effects of pesticide exposure and hidden drivers of PD pathogenesis beyond direct DA neurons damage.
Regarding specific toxin PD-models, decreased Prevotellaceae and increased Lactobacillaceae and Bifidobacteriaceae were found in a rotenone-induced rat model of PD [173], and these changes have been associated with reduced serum ghrelin [177, 180], a gut hormone regulating nigrostriatal dopamine function with the proposed ability to attenuate neurodegeneration in PD [181]. In this aspect, PD patients have been reported as having impaired ghrelin secretion, which may increase the vulnerability of the nigrostriatal neurons and contribute to the development of GI symptoms in PD patients, as ghrelin promotes gastrointestinal motility [245].
An overview of pesticides and the microbiome-gut-brain axis interactions and contributions to the pathogenesis of PD is displayed in Fig. 1.
Fig. 1
Pesticides and the microbiome-gut-brain-axis in PD pathogenesis –convergent pathways. Pesticides exert both direct and indirect effects on the nervous system. Direct mechanisms include toxicity on dopaminergic neurons of the substantia nigra in the CNS and impairment of the ENS, while indirect mechanisms are related to alterations of the gut microbiome with multiple subsequent effects, such as leaky gut (leading to self-perpetuating increased exposure of the ENS and CNS to the pesticides), (neuro)inflammation and metabolic/endocrine disruption (mediated, e.g., by bacterial metabolites); gut microbiota can modify the effects of pesticides by changing their pharmacokinetics. CNS, central nervous system; ENS, enteric nervous system; GIT, gastrointestinal tract; SCFA, short-chain fatty acids.
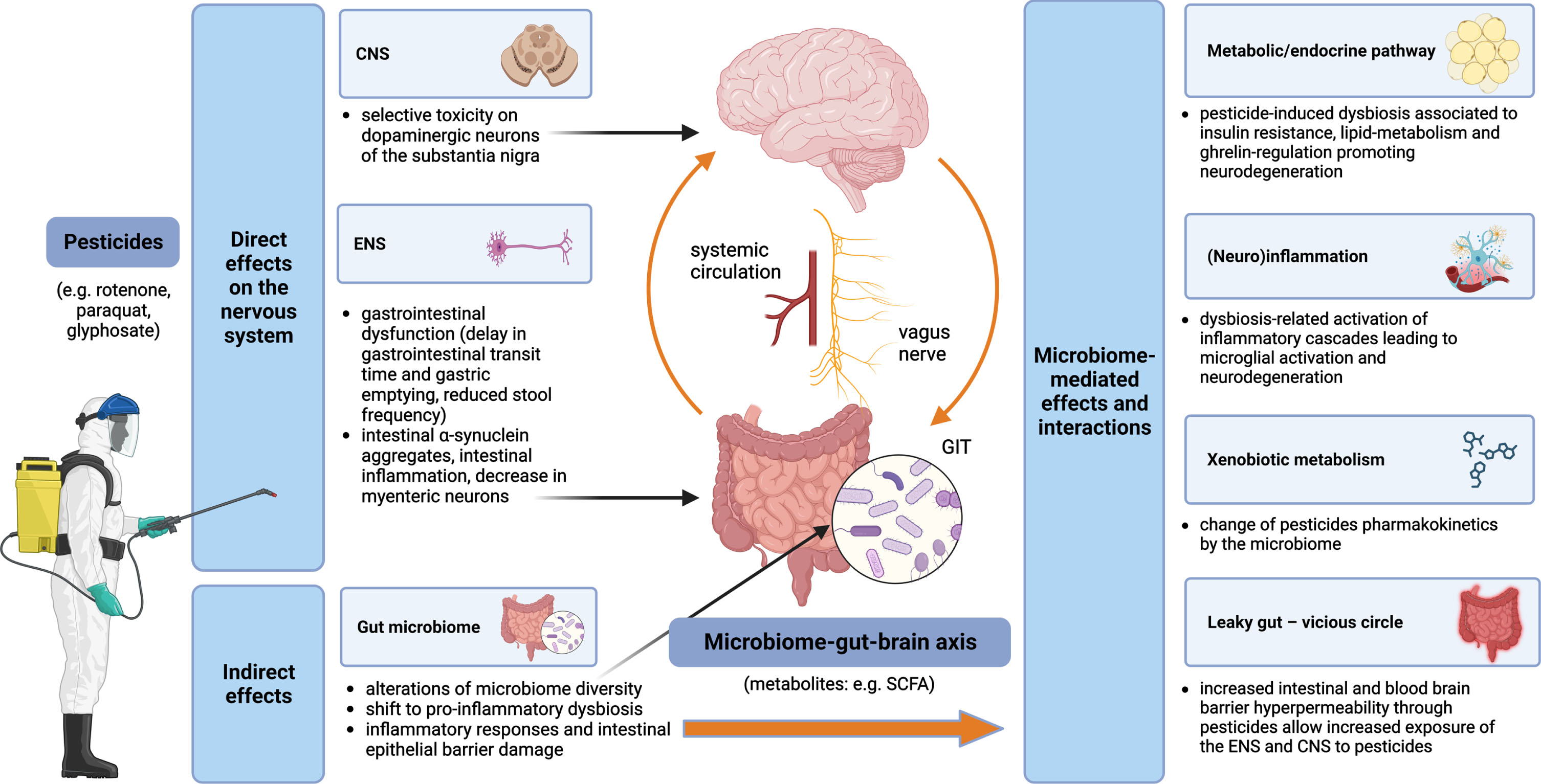
GAPS AND FUTURE DIRECTIONS RELATED TO PESTICIDES AND THE MICROBIOME-GUT-BRAIN AXIS
Brain-first vs. body-first PD
Two basic PD phenotypes have been proposed in regards to the trajectory of disease progression, as introduced in the section on prodromal PD in general, the so-called brain-first PD and body-first PD [132], with the opposite origin site of α-syn misfolding and subsequent spreading (brain-first: descendent trajectory starting in the CNS, body-first: ascendent trajectory starting in the gut—the ENS/PNS). To acknowledge possible oversimplification, this model might not explain the full clinical phenotypic variability of PD (which is currently considered more a spectrum of diseases than just one unit [246]). Moreover, it might not be relevant for genetic forms of PD with almost no or only limited α-syn pathology (including, for example, the PRKN or LRRK2 mutation) and might be of questionable relevance to genetic forms of PD in general (as many have atypical clinical phenotypes, although some might fit into this model; LRRK2-PD patients mostly exhibit a brain-first PD profile, while patients with GBA variants typically resemble a body-first profile, and SNCA-variant carriers are equally distributed between both subtypes [247]). However, it may still be useful in cases of idiopathic PD (accounting for almost 90% of PD worldwide), presenting an interesting research framework to study the disease progression.
It was shown that the body-first group has a greater colon transit time and greater colon volume compared to the brain-first group, indicating more constipation [248]. Thus, a substantial contribution of the microbiome-gut-brain axis to PD pathogenesis from the very beginning seems to be plausible in the body-first subtype. Evidence for specific involvement of either pesticides or the microbiome in the brain-/body-first PD subtypes is currently limited, although some observations have already been made. Interestingly, it was shown that pesticide exposure is more common in PD cases with RBD and symmetric parkinsonism, representing the body-first subtype. This suggests that ingested pesticides may have a stronger propensity to trigger enteric pathology rather than olfactory bulb pathology [249].
As for microbiome changes in PD, the broad heterogeneity of findings has a number of possible reasons [250]; however, brain-/body-first phenotypes were not taken into account and could perhaps explain part of the inconsistencies [251]. It could be expected that PD-related changes in microbiota composition would be more pronounced in the body-first group. Although we do not have such direct evidence yet, as previous studies focused on PD mostly as a single group without differentiating between the proposed subtypes, the first indications come from studies of iRBD patients (as a proxy for prodromal body-first PD). More than 75% of the identified bacteria differing between PD and controls were qualitatively similar to the differences between iRBD and controls [234, 252].
Overall, it seems that both pesticides and microbiome-related contribution to PD pathogenesis is more linked to the body-first phenotype, although these conclusions are still preliminary, and further studies are definitely needed to gain deeper insight into the differences between these two PD subtypes.
Gene-environment interactions
An example of potential gene-environment interactions is the crosstalk between LRRK2 protein and the pesticides rotenone [253–255] and paraquat [256, 257], as well as other environmental toxicants, such as solvent trichloroethylene [258] and manganese [259, 260]. Pathological elevation in LRRK2 kinase activity induced by environmental toxicants resembles LRRK2 gain-of-function mutations; therefore, pharmaceutical inhibition of LRRK2 by small molecule kinase inhibitors [261] could be appropriate after certain exposures [42, 262]. Taken together, it can be hypothesized that LRRK2-mutation carriers might be particularly vulnerable to the above-mentioned pesticides. Another example of gene-environment interaction that promotes risk for PD via alterations in immune responses can be a single nucleotide polymorphism in the major histocompatibility complex class II (MHC-II), which increases susceptibility to PD through the presentation of pathogenic, immunodominant antigens and/or a shift toward a more pro-inflammatory CD4 + T cell response in response to specific environmental exposures, such as pyrethroid exposure [263]. Defective cellular protection against oxidative stress represents another mechanism. PD risk from paraquat exposure was high in individuals with homozygous deletions of the genes encoding glutathione S-transferase [264]. Scavenger capacity also plays a role: butyrylcholinesterase (BChE, a known bioscavenger for pesticides) decreases PD risk, while K-variant BCHE reduces the serum activity of BChE and results in increased PD risk in pesticide-exposed individuals, particularly in case of insecticides, such as organophosphates and carbamates [265].
Genetic background can also influence pesticide-microbiome-host interactions, although findings are still rather scarce. Mutations of the HFE protein (High Fe2 +, a critical human regulator of cellular iron uptake) are associated with increased iron accumulation, including the brain, and the commonly occurring H63D variant is considered as a disease modifier in several neurodegenerative diseases [266]. HFE mutant mice showed reduced expression of tyrosine hydroxylase in the SN; however, they presented with alterations in the gut microbiome profile contrary to PD pattern and were resistant to paraquat toxicity, altogether providing additional support for HFE genotype being a disease modifier for PD as well as a model to study mechanisms of resistance to neurotoxicants [267].
Many gene-environment interactions were studied in isolation. In future research, it will be important to develop comprehensive and systematic approaches to these complex relationships [42], such as studying genetic risk factors modifying pesticide-microbiome interactions in PD.
TARGETING PESTICIDES AND THE MICROBIOME-GUT-BRAIN AXIS: POSSIBILITIES FOR TRANSLATION
Given the current unavailability of causal disease-modifying treatment for PD while facing a Parkinson pandemic, especially in areas with rapid industrialization, it is important to view pesticides, the microbiome and their crosstalk as potential targets for preventive strategies. As the global prevalence of PD is projected to double by 2040 [268], all efforts should be made to decrease the burden through the lens of modifiable factors [5]. Where feasible, PD should be prevented by reducing and in some cases eliminating the use of chemicals known to increase the risk of PD, pesticides in particular [7]. On the other hand, any final call for action has to stand on a solid scientific foundation concerning the causative role of pesticides in PD pathogenesis, keeping in mind the consequences of the potential banning of glyphosate or other pesticides in a global perspective [219]. Fighting neurodegeneration must go hand in hand with fighting starvation in developing countries, so that solving the first problem (by the potential banning of multiple pesticides) does not deepen the second (by subsequent lower food production), especially given that pesticides are just one piece of the mosaic in PD pathogenesis and even banning all of them globally would not lead to the eradication of PD.
Although many mechanisms of pesticide-induced neurodegeneration have already been discovered, a lot is still unknown, posing a challenge for bulletproof large-scale recommendations concerning pesticide use or banning. In a “manifesto” titled as an environmental agenda to prevent PD, De Miranda et al. compiled research priorities from both basic and translational perspectives—to model complex environmental exposure and identify its relevant concentrations and routes of entry, to examine gender differences, gene-environment interactions and modifying effects of microbiome and diet, to track global PD incidence and its change with a special focus on populations with high exposure burden and to develop reliable biological markers of exposure and use them as early as in the prodromal phase [42].
The outright banning of pesticide use is currently not feasible due to food security concerns and the lack of viable alternatives for large-scale replacement [269]. One possible way out and a promising alternative to reduce or replace the use of agrochemicals is the use of biopesticides and genetically modified organisms, microorganisms that target specific pathogens, biochemical pesticides that control insect behavior and plant-incorporated protectants [270–272]. However, there are several challenges for a large-scale utilization of biopesticides to become a reality: their cost-effectiveness, farmers’ awareness, eventual incompatibilities between pesticides and microbial inoculants and safety to non-target organisms (including humans) [269]. Last, but not least, it is important to promote international cooperation to support the monitoring capabilities of developing countries, which usually lack the expertise and resources to implement effective monitoring programs for pesticide contaminants in both drinking water and food [273].
Still, even in the case of potential pesticides banning or an instant global transition to biopesticides, the long latency between these restrictions and any evident protective effect on the prevalence of PD has to be expected. Yuan et al. found high pesticide exposure among US farmers to be related to prodromal features of PD, such as dream-enacting behavior (indicating iRBD) with a time gap up to 40 years, which means the interval between pesticide exposure and the onset of manifest PD can be even up to 5 decades [49, 274], and early adulthood might be an etiological window of particular interest [275]. The results of current changes might take decades to be harvested, yet this fact should not serve as an excuse for resignation, but instead, as a motivation to search for alternative preventive measures that can be applied in the meantime.
Except for global recommendations to decrease the use of pesticides with a known connection to PD, there are some smaller but not unimportant steps to take for those who cannot avoid pesticide exposure. First, the use of protective tools and hygiene practices modified the association between pesticides and PD in epidemiological studies. Neither pesticide was associated with PD among protective glove users [276, 277], and a longer delay between the event of high pesticide exposure and subsequent washing with soap and water further increased the risk of dream-enacting behaviors [49]. Thus, taking personal protection equipment and hygiene seriously should be emphasized in agricultural workers to prevent occupational exposure, and using some forms of personal protection could also be advised to people who live in areas with high pesticide use to lower the environmental burden. Second, new innovative approaches (such as the utilization of N-acetyl cysteine, vitamin C, vitamin E, cyclophosphamide, or probiotics) are proposed for the treatment of acute herbicide poisoning where no proven antidote exists (paraquat, glyphosate) [278]. If the benefit is confirmed and the safety profile is acceptable, it would also be worth considering potential benefits of these approaches in terms of chronic exposure.
The other side of the coin is placing the focus on the microbiome-gut-brain axis and designing future preventive and therapeutic strategies to increase its resistance against pesticides. Concerning dietary habits and PD, higher intake of N-3 polyunsaturated fatty acids (PUFAs) was inversely associated with PD risk (possibly related to the ability of N-3 PUFAs to limit the inflammatory response). Moreover, fat intake modified the associations of PD with the pesticides paraquat and rotenone, suggesting that a diet low in PUFAs or high in saturated fat may increase vulnerability to pesticides and increase PD risk in case of exposure [279]. Developing specific approaches to strengthen the microbiome in PD through exercise, diet modification, prebiotics, probiotics, postbiotics, antimicrobial agents or fecal microbiota transplants is in the scope of several recently published reviews [28, 139, 140, 280, 281]. These strategies could be of relevance, especially in professions and areas where pesticide exposure cannot be avoided, but due to a generally safe profile they might be beneficial also on a large-scale population basis (at least the lifestyle modifications). A summary of potential preventive measures related to both pesticides and microbiome is outlined in Fig. 2.
Fig. 2
Targeting pesticides and the microbiome-gut-brain axis. Multiple measures are at hand to reduce the global burden of PD, aimed at both pesticides and microbiome. Where possible, the use of PD-relevant pesticides should be reduced and replaced by safer alternatives; effective monitoring should control food and water contamination, and protective measures, such as the use of personal protective equipment or new approaches for the treatment of poisoning, should be emphasized where pesticide exposure is inevitable. On the other hand, strategies aimed at strengthening the microbiome could be used on a large scale (general lifestyle modifications, such as exercise and a healthy diet), while more targeted interventions, including the use of pre/pro/postbiotics, antimicrobial agents or fecal microbiota transplants, could be relevant in specific conditions associated with pesticide exposure. PD, Parkinson’s disease; GMO, genetically modified organisms.
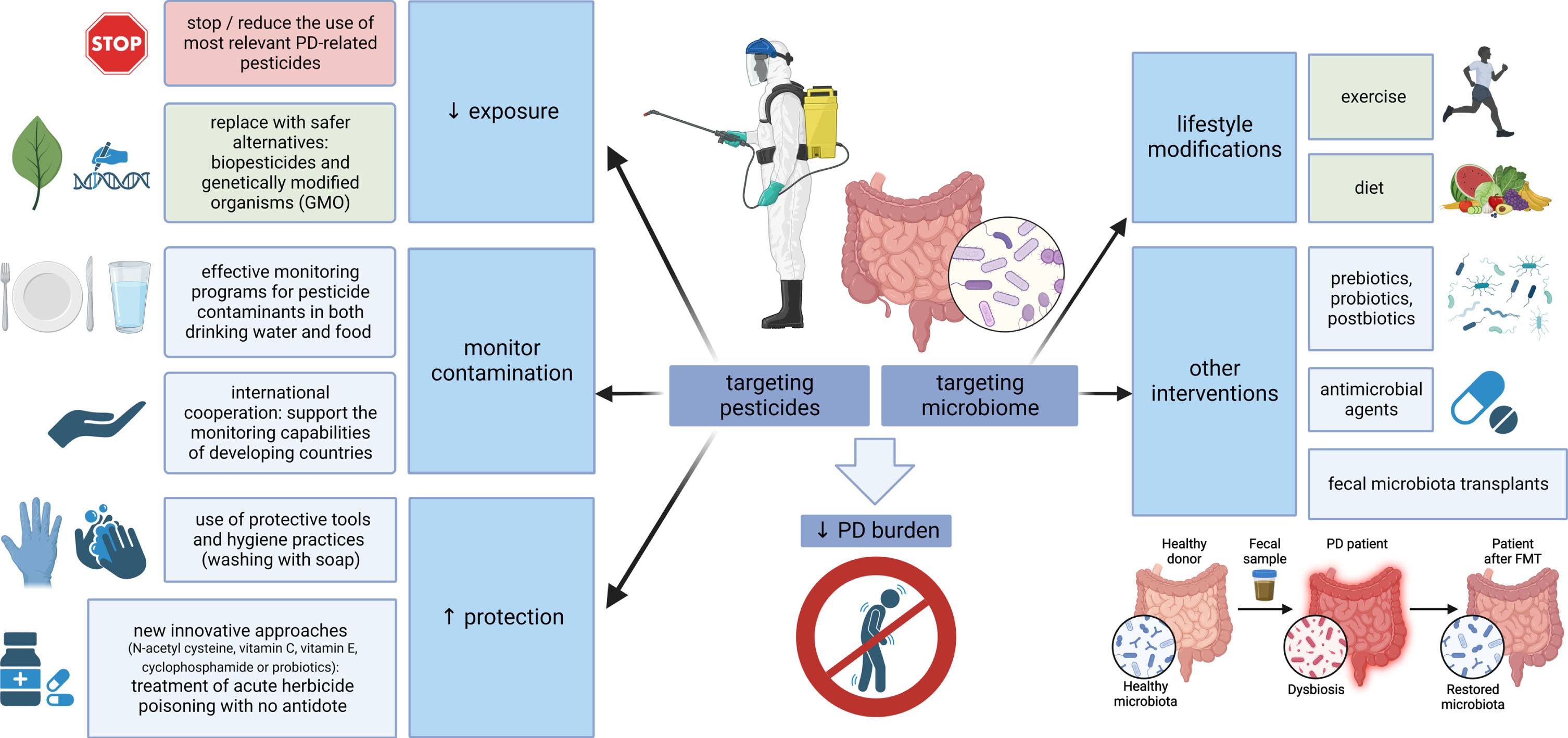
CONCLUSIONS
The increasing global burden of PD draws our attention to potentially modifiable causes that would open the door for preventive measures. Historical evidence of the existence of PD centuries before synthetic pesticides and industrial solvents were manufactured and extensively used argues against their having a sole causative role. However, current epidemiological studies linking chronic occupational or environmental pesticide exposure with higher PD prevalence [282], records of PD development after acute high-dose pesticide intoxication, as well as animal PD models where the disease initiation and progression follows experimental pesticide exposure alone provide strong proof that pesticides may be significant contributors in at least a subset of PD cases.
Although many risk factors and disease modifiers were assessed independently in the past, it is clear today that influences of the environment and lifestyle, the gene-environment and environment-environment interactions are enormously complex and deserve focused interest to untangle hidden convergent pathways and mutual influence on PD pathogenesis. Air pollution emerges as one of newly recognized factors increasing PD risk and acting through multiple similar mechanisms as pesticides: direct neuronal toxicity, induction of systemic inflammation leading to CNS inflammation and alterations in gut physiology and the microbiome [283]. Various environmental factors might act synergistically, as illustrated by the example of paraquat and traumatic brain injury: both increase the risk of PD independently, but combined exposure almost triples the risk of the disease [284, 285].
Pesticide-microbiome crosstalk is an important part of the overall picture. Many effects of both pesticides and microbiome converge in impairing intestinal barrier integrity, leading to gut and systemic inflammation, with microbial metabolites playing an important role in mediating multiple inflammatory and metabolic cascades, finally resulting in an accumulation of misfolded α-syn and neurodegeneration.
A lot is still unknown: the differences between body-/brain-first PD, the complexity of environmental pollution, the search for relevant and reliable biomarkers of toxicant exposure, understanding gender differences, genetic mechanisms of susceptibility and resistance, the multi-omics perspective of microbiome-mediated effects and many others. Importantly, even today we know enough to move one step forward to focus on preventive measures to soften the deleterious impact of pesticides and dysbiosis on the PD burden. Where possible, the use of dangerous pesticides with a clear link to PD, such as paraquat, should be discouraged and replaced by safer alternatives. In the meantime, the use of protective equipment should be emphasized, and new pharmacological or complementary dietary approaches should be considered in populations unable to avoid pesticide exposure. Last but not least: many microbiome-targeted strategies, such as exercise, diet, prebiotics, probiotics, postbiotics, ATB, small molecule drugs, phage therapy or FMT, are emerging. They represent promising tools with a safe profile not only for high-risk agricultural occupations, but also to induce positive changes and strengthen the microbiome in the general population. To conclude, although further research is needed to understand the pathophysiological background better, possible meaningful preventive interventions to fight the neurodegeneration and PD pandemic, such as reducing the use of harmful substances or promoting healthy lifestyle modifications, are at hand, which is hopeful particularly in the conditions of unavailability of causal disease-modifying treatment of PD that we still have to face.
ACKNOWLEDGMENTS
All figures were created with BioRender.com.
FUNDING
This work was supported by Slovak Research and Development Agency under contract no. APVV-22-0279 and by the Slovak Scientific Grant Agency under contract no. VEGA 1/0712/22 for the Slovak site.
CONFLICT OF INTEREST
The authors have no conflict of interest to report.
REFERENCES
[1] | Poewe W , Seppi K , Tanner CM , Halliday GM , Brundin P , Volkmann J , Schrag A-E , Lang AE ((2017) ) Parkinson disease. Nat Rev Dis Primer 3: , 17013. |
[2] | Pan L , Meng L , He M , Zhang Z ((2021) ) Tau in the pathophysiology of Parkinson’s disease. J Mol Neurosci 71: , 2179–2191. |
[3] | Pan L , Li C , Meng L , Tian Y , He M , Yuan X , Zhang G , Zhang Z , Xiong J , Chen G , Zhang Z ((2022) ) Tau accelerates α-synuclein aggregation and spreading in Parkinson’s disease. Brain 145: , 3454–3471. |
[4] | Feigin VL , Abajobir AA , Abate KH , Abd-Allah F , Abdulle AM , Abera SF , Abyu GY , Ahmed MB , Aichour AN , Aichour I ,et al. ((2017) ) Global, regional, and national burden of neurological disorders during 1990–2015: A systematic analysis for the Global Burden of Disease Study 2015. Lancet Neurol 16: , 877–897. |
[5] | Feigin VL , Nichols E , Alam T , Bannick MS , Beghi E , Blake N , Culpepper WJ , Dorsey ER , Elbaz A , Ellenbogen RG , et al. ((2019) ) Global, regional, and national burden of neurological disorders, 1990–2016: A systematic analysis for the Global Burden of Disease Study 2016. Lancet Neurol 18: , 459–480. |
[6] | Ou Z , Pan J , Tang S , Duan D , Yu D , Nong H , Wang Z ((2021) ) Global trends in the incidence, prevalence, and years lived with disability of Parkinson’s disease in 204 countries/territories from 1990 to 2019. Front Public Health 9: , 776847. |
[7] | Dorsey ER , Sherer T , Okun MS , Bloem BR ((2018) ) The emerging evidence of the Parkinson pandemic. J Parkinsons Dis 8: , S3–S8. |
[8] | Allen L ((2017) ) Are we facing a noncommunicable disease pandemic? . J Epidemiol Glob Health 7: , 5–9. |
[9] | GBD 2015 SDG Collaborators ((2016) ) Measuring the health-related Sustainable Development Goals in 188 countries: A baseline analysis from the Global Burden of Disease Study 2015. Lancet 388: , 1813–1850. |
[10] | Pezzoli G , Cereda E ((2013) ) Exposure to pesticides or solvents and risk of Parkinson disease. Neurology 80: , 2035–2041. |
[11] | Weisskopf MG , Weuve J , Nie H , Saint-Hilaire M-H , Sudarsky L , Simon DK , Hersh B , Schwartz J , Wright RO , Hu H ((2010) ) Association of cumulative lead exposure with Parkinson’s disease. Environ Health Perspect 118: , 1609–1613. |
[12] | Goldman SM ((2014) ) Environmental toxins and Parkinson’s disease. Annu Rev Pharmacol Toxicol 54: , 141–164. |
[13] | Muthane UB , Ragothaman M , Gururaj G ((2007) ) Epidemiology of Parkinson’s disease and movement disorders in India: Problems and possibilities. J Assoc Physicians India 55: , 719–724. |
[14] | Azizova TV , Bannikova MV , Grigoryeva ES , Rybkina VL , Hamada N ((2020) ) Occupational exposure to chronic ionizing radiation increases risk of Parkinson’s disease incidence in Russian Mayak workers. Int J Epidemiol 49: , 435–447. |
[15] | Noyce AJ , Bestwick JP , Silveira-Moriyama L , Hawkes CH , Giovannoni G , Lees AJ , Schrag A ((2012) ) Meta-analysis of early nonmotor features and risk factors for Parkinson disease. Ann Neurol 72: , 893–901. |
[16] | Gunnarsson L-G , Bodin L ((2017) ) Parkinson’s disease and occupational exposures: A systematic literature review and meta-analyses. Scand J Work Environ Health 43: , 197–209. |
[17] | Berg D , Postuma RB , Adler CH , Bloem BR , Chan P , Dubois B , Gasser T , Goetz CG , Halliday G , Joseph L , Lang AE , Liepelt-Scarfone I , Litvan I , Marek K , Obeso J , Oertel W , Olanow CW , Poewe W , Stern M , Deuschl G ((2015) ) MDS research criteria for prodromal Parkinson’s disease. Mov Disord 30: , 1600–1611. |
[18] | Heinzel S , Berg D , Gasser T , Chen H , Yao C , Postuma RB , MDS Task Force on the Definition of Parkinson’s Disease ((2019) ) Update of the MDS research criteria for prodromal Parkinson’s disease. Mov Disord 34: , 1464–1470. |
[19] | van der Mark M , Brouwer M , Kromhout H , Nijssen P , Huss A , Vermeulen R ((2012) ) Is pesticide use related to Parkinson disease? Some clues to heterogeneity in study results. Environ Health Perspect 120: , 340–347. |
[20] | Van Maele-Fabry G , Hoet P , Vilain F , Lison D ((2012) ) Occupational exposure to pesticides and Parkinson’s disease: A systematic review and meta-analysis of cohort studies. Environ Int 46: , 30–43. |
[21] | Elbaz A , Moisan F ((2016) ) The scientific bases to consider Parkinson’s disease an occupational disease in agriculture professionals exposed to pesticides in France. J Epidemiol Community Health 70: , 319–321. |
[22] | Kingdom of Sweden v Commission of the European Communities. Directive 91/414/EEC –Plant protection products –Paraquat as an active substance –Marketing authorisation –Authorisation procedure –Protection of human and animal health. Case T-229/04. European Court Reports 2007 II-02437. ECLI identifier: ECLI:EU:T:2007:217. |
[23] | Myung W , Lee G-H , Won H-H , Fava M , Mischoulon D , Nyer M , Kim DK , Heo J-Y , Jeon HJ ((2015) ) Paraquat prohibition and change in the suicide rate and methods in South Korea. PLoS One 10: , e0128980. |
[24] | Huang Y , Zhan H , Bhatt P , Chen S ((2019) ) Paraquat degradation from contaminated environments: Current achievements and perspectives. Front Microbiol 10: , 1754. |
[25] | Arotcarena M-L , Dovero S , Prigent A , Bourdenx M , Camus S , Porras G , Thiolat M-L , Tasselli M , Aubert P , Kruse N , Mollenhauer B , Trigo Damas I , Estrada C , Garcia-Carrillo N , Vaikath NN , El-Agnaf OMA , Herrero MT , Vila M , Obeso JA , Derkinderen P , Dehay B , Bezard E ((2020) ) Bidirectional gut-to-brain and brain-to-gut propagation of synucleinopathy in non-human primates. Brain 143: , 1462–1475. |
[26] | Ghaisas S , Maher J , Kanthasamy A ((2016) ) Gut microbiome in health and disease: Linking the microbiome–gut–brain axis and environmental factors in the pathogenesis of systemic and neurodegenerative diseases. Pharmacol Ther 158: , 52–62. |
[27] | Carabotti M , Scirocco A , Maselli MA , Severi C ((2015) ) The gut-brain axis: Interactions between enteric microbiota, central and enteric nervous systems. Ann Gastroenterol 28: , 203–209. |
[28] | Sun X , Xue L , Wang Z , Xie A ((2022) ) Update to the treatment of Parkinson’s disease based on the gut-brain axis mechanism. Front Neurosci 16: , 878239. |
[29] | Yuan X , Pan Z , Jin C , Ni Y , Fu Z , Jin Y ((2019) ) Gut microbiota: An underestimated and unintended recipient for pesticide-induced toxicity. Chemosphere 227: , 425–434. |
[30] | Sharma T , Sirpu Natesh N , Pothuraju R , Batra SK , Rachagani S ((2023) ) Gut microbiota: A non-target victim of pesticide-induced toxicity. Gut Microbes 15: , 2187578. |
[31] | Matsuzaki R , Gunnigle E , Geissen V , Clarke G , Nagpal J , Cryan JF ((2023) ) Pesticide exposure and the microbiota-gut-brain axis. ISME J 17: , 1153–1166. |
[32] | Gois MFB , Fernández-Pato A , Huss A , Gacesa R , Wijmenga C , Weersma RK , Fu J , Vermeulen RCH , Zhernakova A , Lenters VC , Kurilshikov A ((2023) ) Impact of occupational pesticide exposure on the human gut microbiome. Front Microbiol 14: , 1223120. |
[33] | Langston JW , Ballard P , Tetrud JW , Irwin I ((1983) ) Chronic Parkinsonism in humans due to a product of meperidine-analog synthesis. Science 219: , 979–980. |
[34] | Cicchetti F , Drouin-Ouellet J , Gross RE ((2009) ) Environmental toxins and Parkinson’s disease: What have we learned from pesticide-induced animal models? Trends Pharmacol Sci 30: , 475–483. |
[35] | Markey SP , Johannessen JN , Chiueh CC , Burns RS , Herkenham MA ((1984) ) Intraneuronal generation of a pyridinium metabolite may cause drug-induced parkinsonism. Nature 311: , 464–467. |
[36] | Betarbet R , Sherer TB , MacKenzie G , Garcia-Osuna M , Panov AV , Greenamyre JT ((2000) ) Chronic systemic pesticide exposure reproduces features of Parkinson’s disease. Nat Neurosci 3: , 1301–1306. |
[37] | Cannon JR , Tapias V , Na HM , Honick AS , Drolet RE , Greenamyre JT ((2009) ) A highly reproducible rotenone model of Parkinson’s disease. Neurobiol Dis 34: , 279–290. |
[38] | McCormack AL , Thiruchelvam M , Manning-Bog AB , Thiffault C , Langston JW , Cory-Slechta DA , Di Monte DA ((2002) ) Environmental risk factors and Parkinson’s disease: Selective degeneration of nigral dopaminergic neurons caused by the herbicide paraquat. Neurobiol Dis 10: , 119–127. |
[39] | Brooks AI , Chadwick CA , Gelbard HA , Cory-Slechta DA , Federoff HJ ((1999) ) Paraquat elicited neurobehavioral syndrome caused by dopaminergic neuron loss. Brain Res 823: , 1–10. |
[40] | Thiruchelvam M , Richfield EK , Baggs RB , Tank AW , Cory-Slechta DA ((2000) ) The nigrostriatal dopaminergic system as a preferential target of repeated exposures to combined paraquat and maneb: Implications for Parkinson’s disease. J Neurosci 20: , 9207–9214. |
[41] | Hatcher JM , Richardson JR , Guillot TS , McCormack AL , Di Monte DA , Jones DP , Pennell KD , Miller GW ((2007) ) Dieldrin exposure induces oxidative damage in the mouse nigrostriatal dopamine system. Exp Neurol 204: , 619–630. |
[42] | De Miranda BR , Goldman SM , Miller GW , Greenamyre JT , Dorsey ER ((2022) ) Preventing Parkinson’s disease: An environmental agenda. J Parkinsons Dis 12: , 45–68. |
[43] | Wirdefeldt K , Adami H-O , Cole P , Trichopoulos D , Mandel J ((2011) ) Epidemiology and etiology of Parkinson’s disease: A review of the evidence. Eur J Epidemiol 26: , 1–58. |
[44] | Freire C , Koifman S ((2012) ) Pesticide exposure and Parkinson’s disease: Epidemiological evidence of association. Neurotoxicology 33: , 947–971. |
[45] | Yuan X , Tian Y , Liu C , Zhang Z ((2022) ) Environmental factors in Parkinson’s disease: New insights into the molecular mechanisms. Toxicol Lett 356: , 1–10. |
[46] | Vellingiri B , Chandrasekhar M , Sri Sabari S , Gopalakrishnan AV , Narayanasamy A , Venkatesan D , Iyer M , Kesari K , Dey A ((2022) ) Neurotoxicity of pesticides –A link to neurodegeneration. Ecotoxicol Environ Saf 243: , 113972. |
[47] | Nabi M , Tabassum N ((2022) ) Role of environmental toxicants on neurodegenerative disorders. Front Toxicol 4: , 837579. |
[48] | Chaza C , Sopheak N , Mariam H , David D , Baghdad O , Moomen B ((2018) ) Assessment of pesticide contamination in Akkar groundwater, northern Lebanon. Environ Sci Pollut Res 25: , 14302–14312. |
[49] | Yuan Y , Shrestha S , Luo Z , Li C , Plassman BL , Parks CG , Hofmann JN , Beane Freeman LE , Sandler DP , Chen H ((2022) ) High pesticide exposure events and dream-enacting behaviors among US farmers. Mov Disord 37: , 962–971. |
[50] | Bhatt MH , Elias MA , Mankodi AK ((1999) ) Acute and reversible parkinsonism due to organophosphate pesticide intoxication: Five cases. Neurology 52: , 1467–1471. |
[51] | McKnight S , Hack N ((2020) ) Toxin-Induced Parkinsonism. Neurol Clin 38: , 853–865. |
[52] | Weissbach A , Wittke C , Kasten M , Klein C ((2019) ) “Atypical” Parkinson’s disease –genetic. Int Rev Neurobiol 149: , 207–235. |
[53] | Gasser T ((2015) ) Usefulness of genetic testing in PD and PD trials: A balanced review. J Parkinsons Dis 5: , 209–215. |
[54] | Eriguchi M , Iida K , Ikeda S , Osoegawa M , Nishioka K , Hattori N , Nagayama H , Hara H ((2019) ) Parkinsonism relating to intoxication with glyphosate. Intern Med 58: , 1935–1938. |
[55] | Barbosa ER , Leiros da Costa MD , Bacheschi LA , Scaff M , Leite CC ((2001) ) Parkinsonism after glycine-derivate exposure. Mov Disord 16: , 565–568. |
[56] | Caballero M , Amiri S , Denney JT , Monsivais P , Hystad P , Amram O ((2018) ) Estimated residential exposure to agricultural chemicals and premature mortality by Parkinson’s disease in Washington state. Int J Environ Res Public Health 15: , 2885. |
[57] | Wang G , Fan X-N , Tan Y-Y , Cheng Q , Chen S-D ((2011) ) Parkinsonism after chronic occupational exposure to glyphosate. Parkinsonism Relat Disord 17: , 486–487. |
[58] | Paul KC , Krolewski RC , Lucumi Moreno E , Blank J , Holton KM , Ahfeldt T , Furlong M , Yu Y , Cockburn M , Thompson LK , Kreymerman A , Ricci-Blair EM , Li YJ , Patel HB , Lee RT , Bronstein J , Rubin LL , Khurana V , Ritz B ((2023) ) A pesticide and iPSC dopaminergic neuron screen identifies and classifies Parkinson-relevant pesticides. Nat Commun 14: , 2803. |
[59] | Saravanan KS , Sindhu KM , Mohanakumar KP ((2005) ) Acute intranigral infusion of rotenone in rats causes progressive biochemical lesions in the striatum similar to Parkinson’s disease. Brain Res 1049: , 147–155. |
[60] | Moretto A , Colosio C ((2011) ) Biochemical and toxicological evidence of neurological effects of pesticides: The example of Parkinson’s disease. Neurotoxicology 32: , 383–391. |
[61] | Arnold B , Cassady SJ , VanLaar VS , Berman SB ((2011) ) Integrating multiple aspects of mitochondrial dynamics in neurons: Age-related differences and dynamic changes in a chronic rotenone model. Neurobiol Dis 41: , 189–200. |
[62] | Chu CT , Ji J , Dagda RK , Jiang JF , Tyurina YY , Kapralov AA , Tyurin VA , Yanamala N , Shrivastava IH , Mohammadyani D , Qiang Wang KZ , Zhu J , Klein-Seetharaman J , Balasubramanian K , Amoscato AA , Borisenko G , Huang Z , Gusdon AM , Cheikhi A , Steer EK , Wang R , Baty C , Watkins S , Bahar I , Bayır H , Kagan VE ((2013) ) Cardiolipin externalization to the outer mitochondrial membrane acts as an elimination signal for mitophagy in neuronal cells. Nat Cell Biol 15: , 1197–1205. |
[63] | Johnson ME , Bobrovskaya L ((2015) ) An update on the rotenone models of Parkinson’s disease: Their ability to reproduce the features of clinical disease and model gene–environment interactions. NeuroToxicology 46: , 101–116. |
[64] | Zeng X-S , Geng W-S , Jia J-J ((2018) ) Neurotoxin-induced animal models of Parkinson disease: Pathogenic mechanism and assessment. ASN Neuro 10: , 1759091418777438. |
[65] | Martella G , Madeo G , Maltese M , Vanni V , Puglisi F , Ferraro E , Schirinzi T , Valente EM , Bonanni L , Shen J , Mandolesi G , Mercuri NB , Bonsi P , Pisani A ((2016) ) Exposure to low-dose rotenone precipitates synaptic plasticity alterations in PINK1 heterozygous knockout mice. Neurobiol Dis 91: , 21–36. |
[66] | Wu F , Wang Z , Gu J-H , Ge J-B , Liang Z-Q , Qin Z-H ((2013) ) p38MAPK/p53-Mediated Bax induction contributes to neurons degeneration in rotenone-induced cellular and rat models of Parkinson’s disease. Neurochem Int 63: , 133–140. |
[67] | Feng J ((2006) ) Microtubule: A common target for parkin and Parkinson’s disease toxin. Neuroscientist 12: , 469–476. |
[68] | Mastroberardino PG , Hoffman EK , Horowitz MP , Betarbet R , Taylor G , Cheng D , Na HM , Gutekunst C-A , Gearing M , Trojanowski JQ , Anderson M , Chu CT , Peng J , Greenamyre JT ((2009) ) A novel transferrin/TfR2-mediated mitochondrial iron transport system is disrupted in Parkinson’s disease. Neurobiol Dis 34: , 417–431. |
[69] | Ibarra-Gutiérrez MT , Serrano-García N , Orozco-Ibarra M ((2023) ) Rotenone-induced model of Parkinson’s disease: Beyond mitochondrial complex I inhibition. Mol Neurobiol 60: , 1929–1948. |
[70] | Lawana V , Cannon JR (2020) Chapter Five –Rotenone neurotoxicity: Relevance to Parkinson’s disease. In Advances in Neurotoxicology, Aschner M, Costa LG, eds. Academic Press, pp. 209–254. |
[71] | Höglinger GU , Féger J , Prigent A , Michel PP , Parain K , Champy P , Ruberg M , Oertel WH , Hirsch EC ((2003) ) Chronic systemic complex I inhibition induces a hypokinetic multisystem degeneration in rats. J Neurochem 84: , 491–502. |
[72] | Elgayar SAM , Abdel-Hafez AAM , Gomaa AMS , Elsherif R ((2018) ) Vulnerability of glia and vessels of rat substantia nigra in rotenone Parkinson model. Ultrastruct Pathol 42: , 181–192. |
[73] | Kim S , Pajarillo E , Nyarko-Danquah I , Aschner M , Lee E ((2023) ) Role of astrocytes in Parkinson’s disease associated with genetic mutations and neurotoxicants. Cells 12: , 622. |
[74] | Gao H-M , Hong J-S , Zhang W , Liu B ((2002) ) Distinct role for microglia in rotenone-induced degeneration of dopaminergic neurons. J Neurosci 22: , 782–790. |
[75] | Sherer TB , Betarbet R , Kim JH , Greenamyre JT ((2003) ) Selective microglial activation in the rat rotenone model of Parkinson’s disease. Neurosci Lett 341: , 87–90. |
[76] | Castello PR , Drechsel DA , Patel M ((2007) ) Mitochondria are a major source of paraquat-induced reactive oxygen species production in the brain. J Biol Chem 282: , 14186–14193. |
[77] | Stojkovska I , Wagner BM , Morrison BE ((2015) ) Parkinson’s disease and enhanced inflammatory response. Exp Biol Med 240: , 1387–1395. |
[78] | Janda E , Lascala A , Carresi C , Parafati M , Aprigliano S , Russo V , Savoia C , Ziviani E , Musolino V , Morani F , Isidoro C , Mollace V ((2015) ) Parkinsonian toxin-induced oxidative stress inhibits basal autophagy in astrocytes via NQO2/quinone oxidoreductase 2: Implications for neuroprotection. Autophagy 11: , 1063–1080. |
[79] | Chinta SJ , Woods G , Demaria M , Rane A , Zou Y , McQuade A , Rajagopalan S , Limbad C , Madden DT , Campisi J , Andersen JK ((2018) ) Cellular senescence is induced by the environmental neurotoxin paraquat and contributes to neuropathology linked to Parkinson’s disease. Cell Rep 22: , 930–940. |
[80] | Wills J , Credle J , Oaks AW , Duka V , Lee J-H , Jones J , Sidhu A ((2012) ) Paraquat, but not maneb, induces synucleinopathy and tauopathy in striata of mice through inhibition of proteasomal and autophagic pathways. PLoS One 7: , e30745. |
[81] | Musgrove RE , Helwig M , Bae E-J , Aboutalebi H , Lee S-J , Ulusoy A , Monte DAD ((2019) ) Oxidative stress in vagal neurons promotes parkinsonian pathology and intercellular α-synuclein transfer. J Clin Invest 129: , 3738–3753. |
[82] | Desplats P , Patel P , Kosberg K , Mante M , Patrick C , Rockenstein E , Fujita M , Hashimoto M , Masliah E ((2012) ) Combined exposure to Maneb and Paraquat alters transcriptional regulation of neurogenesis-related genes in mice models of Parkinson’s disease. Mol Neurodegener 7: , 49. |
[83] | Thrash B , Uthayathas S , Karuppagounder SS , Suppiramaniam V , Dhanasekaran M ((2007) ) Paraquat and maneb induced neurotoxicity. Proc West Pharmacol Soc 50: , 31–42. |
[84] | Zhang J , Fitsanakis VA , Gu G , Jing D , Ao M , Amarnath V , Montine TJ ((2003) ) Manganese ethylene-bis-dithiocarbamate and selective dopaminergic neurodegeneration in rat: A link through mitochondrial dysfunction. J Neurochem 84: , 336–346. |
[85] | Barlow BK , Cory-Slechta DA , Richfield EK , Thiruchelvam M ((2007) ) The gestational environment and Parkinson’s disease: Evidence for neurodevelopmental origins of a neurodegenerative disorder. Reprod Toxicol 23: , 457–470. |
[86] | Hou L , Zhang C , Wang K , Liu X , Wang H , Che Y , Sun F , Zhou X , Zhao X , Wang Q ((2017) ) Paraquat and maneb co-exposure induces noradrenergic locus coeruleus neurodegeneration through NADPH oxidase-mediated microglial activation. Toxicology 380: , 1–10. |
[87] | Madani NA , Carpenter DO ((2022) ) Effects of glyphosate and glyphosate-based herbicides like RoundupTM on the mammalian nervous system: A review. Environ Res 214: , 113933. |
[88] | International Agency for Research on Cancer (2015) IARC Monographs Volume 112: Evaluation of five organophosphate insecticides and herbicides. World Health Organ Lyon. |
[89] | Martínez M-A , Ares I , Rodríguez J-L , Martínez M , Martínez-Larrañaga M-R , Anadón A ((2018) ) Neurotransmitter changes in rat brain regions following glyphosate exposure. Environ Res 161: , 212–219. |
[90] | El-Shenawy NS ((2009) ) Oxidative stress responses of rats exposed to Roundup and its active ingredient glyphosate. Environ Toxicol Pharmacol 28: , 379–385. |
[91] | Cattani D , Cesconetto PA , Tavares MK , Parisotto EB , De Oliveira PA , Rieg CEH , Leite MC , Prediger RDS , Wendt NC , Razzera G , Filho DW , Zamoner A ((2017) ) Developmental exposure to glyphosate-based herbicide and depressive-like behavior in adult offspring: Implication of glutamate excitotoxicity and oxidative stress. Toxicology 387: , 67–80. |
[92] | Gui Y , Fan X , Wang H , Wang G , Chen S ((2012) ) Glyphosate induced cell death through apoptotic and autophagic mechanisms. Neurotoxicol Teratol 34: , 344–349. |
[93] | Pu Y , Chang L , Qu Y , Wang S , Tan Y , Wang X , Zhang J , Hashimoto K ((2020) ) Glyphosate exposure exacerbates the dopaminergic neurotoxicity in the mouse brain after repeated administration of MPTP. Neurosci Lett 730: , 135032. |
[94] | Saeedi Saravi SS , Dehpour AR ((2016) ) Potential role of organochlorine pesticides in the pathogenesis of neurodevelopmental, neurodegenerative, and neurobehavioral disorders: A review. Life Sci 145: , 255–264. |
[95] | Pessah IN , Lein PJ , Seegal RF , Sagiv SK ((2019) ) Neurotoxicity of polychlorinated biphenyls and related organohalogens. Acta Neuropathol (Berl) 138: , 363–387. |
[96] | Kanthasamy AG , Kitazawa M , Kanthasamy A , Anantharam V ((2005) ) Dieldrin-induced neurotoxicity: Relevance to Parkinson’s disease pathogenesis. Neurotoxicology 26: , 701–719. |
[97] | Sun F , Anantharam V , Latchoumycandane C , Kanthasamy A , Kanthasamy AG ((2005) ) Dieldrin induces ubiquitin-proteasome dysfunction in α-synuclein overexpressing dopaminergic neuronal cells and enhances susceptibility to apoptotic cell death. J Pharmacol Exp Ther 315: , 69–79. |
[98] | Song C , Kanthasamy A , Anantharam V , Sun F , Kanthasamy AG ((2010) ) Environmental neurotoxic pesticide increases histone acetylation to promote apoptosis in dopaminergic neuronal cells: Relevance to epigenetic mechanisms of neurodegeneration. Mol Pharmacol 77: , 621–632. |
[99] | Kochmanski J , VanOeveren SE , Patterson JR , Bernstein AI ((2019) ) Developmental dieldrin exposure alters DNA methylation at genes related to dopaminergic neuron development and Parkinson’s disease in mouse midbrain. Toxicol Sci 169: , 593–607. |
[100] | Gasmi S , Rouabhi R , Kebieche M , Boussekine S , Salmi A , Toualbia N , Taib C , Bouteraa Z , Chenikher H , Henine S , Djabri B ((2017) ) Effects of Deltamethrin on striatum and hippocampus mitochondrial integrity and the protective role of Quercetin in rats. Environ Sci Pollut Res 24: , 16440–16457. |
[101] | Mohammadi H , Ghassemi-Barghi N , Malakshah O , Ashari S ((2019) ) Pyrethroid exposure and neurotoxicity: A mechanistic approach. Arh Hig Rada Toksikol 70: , 74–89. |
[102] | Wan F , Yu T , Hu J , Yin S , Li Y , Kou L , Chi X , Wu J , Sun Y , Zhou Q , Zou W , Zhang Z , Wang T ((2022) ) The pyrethroids metabolite 3-phenoxybenzoic acid induces dopaminergic degeneration. Sci Total Environ 838: , 156027. |
[103] | Costa LG ((2015) ) The neurotoxicity of organochlorine and pyrethroid pesticides. Handb Clin Neurol 131: , 135–148. |
[104] | Travagli RA , Browning KN , Camilleri M ((2020) ) Parkinson disease and the gut: New insights into pathogenesis and clinical relevance. Nat Rev Gastroenterol Hepatol 17: , 673–685. |
[105] | Anselmi L , Toti L , Bove C , Hampton J , Travagli RA ((2017) ) A nigro-vagal pathway controls gastric motility and is affected in a rat model of parkinsonism. Gastroenterology 153: , 1581–1593. |
[106] | Drolet RE , Cannon JR , Montero L , Greenamyre JT ((2009) ) Chronic rotenone exposure reproduces Parkinson’s disease gastrointestinal neuropathology. Neurobiol Dis 36: , 96–102. |
[107] | Perez-Pardo P , Dodiya HB , Broersen LM , Douna H , van Wijk N , Lopes da Silva S , Garssen J , Keshavarzian A , Kraneveld AD ((2018) ) Gut-brain and brain-gut axis in Parkinson’s disease models: Effects of a uridine and fish oil diet. Nutr Neurosci 21: , 391–402. |
[108] | Cannon JR , Greenamyre JT ((2010) ) Neurotoxic in vivo models of Parkinson’s disease recent advances. Prog Brain Res 184: , 17–33. |
[109] | Greene JG , Noorian AR , Srinivasan S ((2009) ) Delayed gastric emptying and enteric nervous system dysfunction in the rotenone model of Parkinson’s disease. Exp Neurol 218: , 154–161. |
[110] | Tasselli M , Chaumette T , Paillusson S , Monnet Y , Lafoux A , Huchet-Cadiou C , Aubert P , Hunot S , Derkinderen P , Neunlist M ((2013) ) Effects of oral administration of rotenone on gastrointestinal functions in mice. Neurogastroenterol Motil 25: , e183–193. |
[111] | Pan-Montojo F , Schwarz M , Winkler C , Arnhold M , O’Sullivan GA , Pal A , Said J , Marsico G , Verbavatz J-M , Rodrigo-Angulo M , Gille G , Funk RHW , Reichmann H ((2012) ) Environmental toxins trigger PD-like progression via increased alpha-synuclein release from enteric neurons in mice. Sci Rep 2: , 898. |
[112] | Clairembault T , Leclair-Visonneau L , Neunlist M , Derkinderen P ((2015) ) Enteric glial cells: New players in Parkinson’s disease? Mov Disord 30: , 494–498. |
[113] | Benvenuti L , D’Antongiovanni V , Pellegrini C , Antonioli L , Bernardini N , Blandizzi C , Fornai M ((2020) ) Enteric glia at the crossroads between intestinal immune system and epithelial barrier: Implications for Parkinson disease. Int J Mol Sci 21: , 9199. |
[114] | Sherer TB , Richardson JR , Testa CM , Seo BB , Panov AV , Yagi T , Matsuno-Yagi A , Miller GW , Greenamyre JT ((2007) ) Mechanism of toxicity of pesticides acting at complex I: Relevance to environmental etiologies of Parkinson’s disease. J Neurochem 100: , 1469–1479. |
[115] | Palanisamy BN , Sarkar S , Malovic E , Samidurai M , Charli A , Zenitsky G , Jin H , Anantharam V , Kanthasamy A , Kanthasamy AG ((2022) ) Environmental neurotoxic pesticide exposure induces gut inflammation and enteric neuronal degeneration by impairing enteric glial mitochondrial function in pesticide models of Parkinson’s disease: Potential relevance to gut-brain axis inflammation in Parkinson’s disease pathogenesis. Int J Biochem Cell Biol 147: , 106225. |
[116] | Naudet N , Antier E , Gaillard D , Morignat E , Lakhdar L , Baron T , Bencsik A ((2017) ) Oral exposure to paraquat triggers earlier expression of phosphorylated α-synuclein in the enteric nervous system of A53T mutant human α-synuclein transgenic mice. J Neuropathol Exp Neurol 76: , 1046–1057. |
[117] | Bove C , Coleman FH , Travagli RA ((2019) ) Characterization of the basic membrane properties of neurons of the rat dorsal motor nucleus of the vagus in paraquat-induced models of parkinsonism. Neuroscience 418: , 122–132. |
[118] | Bove C , Anselmi L , Travagli RA ((2019) ) Altered gastric tone and motility response to brain-stem dopamine in a rat model of parkinsonism. Am J Physiol Gastrointest Liver Physiol 317: , G1–G7. |
[119] | Wang KD , Zhang BY , Zhang BF , Huang M ((2022) ) Preliminary study on time-dependent changes of intestinal tract and brain-gut axis in mice model of Parkinson’s disease induced by paraquat. Chin J Ind Hyg Occup Dis 40: , 161–169. |
[120] | Rueda-Ruzafa L , Cruz F , Roman P , Cardona D ((2019) ) Gut microbiota and neurological effects of glyphosate. Neurotoxicology 75: , 1–8. |
[121] | Palus K , Bulc M , Całka J ((2022) ) Glyphosate affects the neurochemical phenotype of the intramural neurons in the duodenum in the pig. Neurogastroenterol Motil 35: , e14507. |
[122] | Braak H , Rüb U , Gai WP , Del Tredici K ((2003) ) Idiopathic Parkinson’s disease: Possible routes by which vulnerable neuronal types may be subject to neuroinvasion by an unknown pathogen. J Neural Transm 110: , 517–536. |
[123] | Hawkes CH , Del Tredici K , Braak H ((2007) ) Parkinson’s disease: A dual-hit hypothesis. Neuropathol Appl Neurobiol 33: , 599–614. |
[124] | Pont-Sunyer C , Hotter A , Gaig C , Seppi K , Compta Y , Katzenschlager R , Mas N , Hofeneder D , Brücke T , Bayés A , Wenzel K , Infante J , Zach H , Pirker W , Posada IJ , Álvarez R , Ispierto L , De Fàbregues O , Callén A , Palasí A , Aguilar M , Martí MJ , Valldeoriola F , Salamero M , Poewe W , Tolosa E ((2015) ) The onset of nonmotor symptoms in Parkinson’s disease (the ONSET PD study). Mov Disord 30: , 229–237. |
[125] | Poirier A-A , Aubé B , Côté M , Morin N , Di Paolo T , Soulet D ((2016) ) Gastrointestinal dysfunctions in Parkinson’s disease: Symptoms and treatments. Parkinsons Dis 2016: , e6762528. |
[126] | Challis C , Hori A , Sampson TR , Yoo BB , Challis RC , Hamilton AM , Mazmanian SK , Volpicelli-Daley LA , Gradinaru V ((2020) ) Gut-seeded α-synuclein fibrils promote gut dysfunction and brain pathology specifically in aged mice. Nat Neurosci 23: , 327–336. |
[127] | Stokholm MG , Danielsen EH , Hamilton-Dutoit SJ , Borghammer P ((2016) ) Pathological α-synuclein in gastrointestinal tissues from prodromal Parkinson disease patients. Ann Neurol 79: , 940–949. |
[128] | Bu J , Liu J , Liu K , Wang Z ((2019) ) Diagnostic utility of gut α-synuclein in Parkinson’s disease: A systematic review and meta-analysis. Behav Brain Res 364: , 340–347. |
[129] | Schaeffer E , Kluge A , Böttner M , Zunke F , Cossais F , Berg D , Arnold P ((2020) ) Alpha synuclein connects the gut-brain axis in Parkinson’s disease patients –a view on clinical aspects, cellular pathology and analytical methodology. Front Cell Dev Biol 8: , 573696. |
[130] | Braak H , Del Tredici K ((2008) ) Invited Article: Nervous system pathology in sporadic Parkinson disease. Neurology 70: , 1916–1925. |
[131] | Hawkes CH , Del Tredici K , Braak H ((2009) ) Parkinson’s disease: The dual hit theory revisited. Ann N Y Acad Sci 1170: , 615–622. |
[132] | Borghammer P , Van Den Berge N ((2019) ) Brain-first versus gut-first Parkinson’s disease: A hypothesis. J Parkinsons Dis 9: , S281–S295. |
[133] | Borghammer P ((2021) ) The α-synuclein origin and connectome model (SOC Model) of Parkinson’s disease: Explaining motor asymmetry, non-motor phenotypes, and cognitive decline. J Parkinsons Dis 11: , 455–474. |
[134] | Sampson TR ((2022) ) Introduction: Unraveling the complex contributions of indigenous microbes to neurological health and disease. Int Rev Neurobiol 167: xi–xvi. |
[135] | Heintz-Buschart A , Wilmes P ((2018) ) Human gut microbiome: Function matters. Trends Microbiol 26: , 563–574. |
[136] | Ma Q , Xing C , Long W , Wang HY , Liu Q , Wang R-F ((2019) ) Impact of microbiota on central nervous system and neurological diseases: The gut-brain axis. J Neuroinflammation 16: , 53. |
[137] | Suganya K , Koo B-S ((2020) ) Gut-brain axis: Role of gut microbiota on neurological disorders and how probiotics/prebiotics beneficially modulate microbial and immune pathways to improve brain functions. Int J Mol Sci 21: , E7551. |
[138] | Gubert C , Gasparotto J , Morais LH ((2022) ) Convergent pathways of the gut microbiota–brain axis and neurodegenerative disorders. Gastroenterol Rep 10: , goac017. |
[139] | Tan AH , Lim SY , Lang AE ((2022) ) The microbiome-gut-brain axis in Parkinson disease –from basic research to the clinic. Nat Rev Neurol 18: , 476–495. |
[140] | Varesi A , Campagnoli LIM , Fahmideh F , Pierella E , Romeo M , Ricevuti G , Nicoletta M , Chirumbolo S , Pascale A ((2022) ) The interplay between gut microbiota and Parkinson’s disease: Implications on diagnosis and treatment. Int J Mol Sci 23: , 12289. |
[141] | Ryman S , Vakhtin AA , Richardson SP , Lin HC ((2023) ) Microbiome-gut-brain dysfunction in prodromal and symptomatic Lewy body diseases. J Neurol 270: , 746–758. |
[142] | Schwiertz A , Spiegel J , Dillmann U , Grundmann D , Bürmann J , Faßbender K , Schäfer K-H , Unger MM ((2018) ) Fecal markers of intestinal inflammation and intestinal permeability are elevated in Parkinson’s disease. Parkinsonism Relat Disord 50: , 104–107. |
[143] | Houser MC , Chang J , Factor SA , Molho ES , Zabetian CP , Hill-Burns EM , Payami H , Hertzberg VS , Tansey MG ((2018) ) Stool immune profiles evince gastrointestinal inflammation in Parkinson’s disease. Mov Disord 33: , 793–804. |
[144] | Perez-Pardo P , Dodiya HB , Engen PA , Forsyth CB , Huschens AM , Shaikh M , Voigt RM , Naqib A , Green SJ , Kordower JH , Shannon KM , Garssen J , Kraneveld AD , Keshavarzian A ((2019) ) Role of TLR4 in the gut-brain axis in Parkinson’s disease: A translational study from men to mice. Gut 68: , 829–843. |
[145] | Caputi V , Giron MC ((2018) ) Microbiome-gut-brain axis and toll-like receptors in Parkinson’s disease. Int J Mol Sci 19: , 1689. |
[146] | Burgueño JF , Abreu MT ((2020) ) Epithelial Toll-like receptors and their role in gut homeostasis and disease. Nat Rev Gastroenterol Hepatol 17: , 263–278. |
[147] | Kouli A , Horne CB , Williams-Gray CH ((2019) ) Toll-like receptors and their therapeutic potential in Parkinson’s disease and α-synucleinopathies. Brain Behav Immun 81: , 41–51. |
[148] | Devos D , Lebouvier T , Lardeux B , Biraud M , Rouaud T , Pouclet H , Coron E , Bruley des Varannes S , Naveilhan P , Nguyen J-M , Neunlist M , Derkinderen P ((2013) ) Colonic inflammation in Parkinson’s disease. Neurobiol Dis 50: , 42–48. |
[149] | Clairembault T , Kamphuis W , Leclair-Visonneau L , Rolli-Derkinderen M , Coron E , Neunlist M , Hol EM , Derkinderen P ((2014) ) Enteric GFAP expression and phosphorylation in Parkinson’s disease. J Neurochem 130: , 805–815. |
[150] | Hor JW , Lim S-Y , Khor ES , Chong KK , Song SL , Ibrahim NM , Teh CSJ , Chong CW , Hilmi IN , Tan AH ((2022) ) Fecal calprotectin in Parkinson’s disease and multiple system atrophy. J Mov Disord 15: , 106–114. |
[151] | Stolzenberg E , Berry D , Yang D , Lee EY , Kroemer A , Kaufman S , Wong GCL , Oppenheim JJ , Sen S , Fishbein T , Bax A , Harris B , Barbut D , Zasloff MA ((2017) ) A role for neuronal alpha-synuclein in gastrointestinal immunity. J Innate Immun 9: , 456–463. |
[152] | Allen Reish HE , Standaert DG ((2015) ) Role of α-synuclein in inducing innate and adaptive immunity in Parkinson disease. J Parkinsons Dis 5: , 1–19. |
[153] | Forsyth CB , Shannon KM , Kordower JH , Voigt RM , Shaikh M , Jaglin JA , Estes JD , Dodiya HB , Keshavarzian A ((2011) ) Increased intestinal permeability correlates with sigmoid mucosa alpha-synuclein staining and endotoxin exposure markers in early Parkinson’s disease. PloS One 6: , e28032. |
[154] | Kelly LP , Carvey PM , Keshavarzian A , Shannon KM , Shaikh M , Bakay RAE , Kordower JH ((2014) ) Progression of intestinal permeability changes and alpha-synuclein expression in a mouse model of Parkinson’s disease. Mov Disord 29: , 999–1009. |
[155] | Wang W , Nguyen LTT , Burlak C , Chegini F , Guo F , Chataway T , Ju S , Fisher OS , Miller DW , Datta D , Wu F , Wu C-X , Landeru A , Wells JA , Cookson MR , Boxer MB , Thomas CJ , Gai WP , Ringe D , Petsko GA , Hoang QQ ((2016) ) Caspase-1 causes truncation and aggregation of the Parkinson’s disease-associated protein α-synuclein. Proc Natl Acad Sci U S A 113: , 9587–9592. |
[156] | Grathwohl S , Quansah E , Maroof N , Steiner JA , Spycher L , Benmansour F , Duran-Pacheco G , Siebourg-Polster J , Oroszlan-Szovik K , Remy H , Haenggi M , Stawiski M , Selhausen M , Mailver P , Wolfert A , Emrich T , Madaj Z , Su A , Galvis MLE , Mueller C , Herrmann A , Brundin P , Britschgi M ((2021) ) Specific immune modulation of experimental colitis drives enteric alpha-synuclein accumulation and triggers age-related Parkinson-like brain pathology. Free Neuropathol 2: , 13–13. |
[157] | Resnikoff H , Metzger JM , Lopez M , Bondarenko V , Mejia A , Simmons HA , Emborg ME ((2019) ) Colonic inflammation affects myenteric alpha-synuclein in nonhuman primates. J Inflamm Res 12: , 113–126. |
[158] | Prigent A , Lionnet A , Durieu E , Chapelet G , Bourreille A , Neunlist M , Rolli-Derkinderen M , Derkinderen P ((2019) ) Enteric alpha-synuclein expression is increased in Crohn’s disease. Acta Neuropathol (Berl) 137: , 359–361. |
[159] | Codolo G , Plotegher N , Pozzobon T , Brucale M , Tessari I , Bubacco L , de Bernard M ((2013) ) Triggering of inflammasome by aggregated α-synuclein, an inflammatory response in synucleinopathies. PloS One 8: , e55375. |
[160] | Tan E-K , Chao Y-X , West A , Chan L-L , Poewe W , Jankovic J ((2020) ) Parkinson disease and the immune system –associations, mechanisms and therapeutics. Nat Rev Neurol 16: , 303–318. |
[161] | Chen SG , Stribinskis V , Rane MJ , Demuth DR , Gozal E , Roberts AM , Jagadapillai R , Liu R , Choe K , Shivakumar B , Son F , Jin S , Kerber R , Adame A , Masliah E , Friedland RP ((2016) ) Exposure to the functional bacterial amyloid protein curli enhances alpha-synuclein aggregation in aged Fischer 344 rats and Caenorhabditis elegans. Sci Rep 6: , 34477. |
[162] | Sampson TR , Challis C , Jain N , Moiseyenko A , Ladinsky MS , Shastri GG , Thron T , Needham BD , Horvath I , Debelius JW , Janssen S , Knight R , Wittung-Stafshede P , Gradinaru V , Chapman M , Mazmanian SK ((2020) ) A gut bacterial amyloid promotes α-synuclein aggregation and motor impairment in mice. eLife 9: , e53111. |
[163] | Sampson TR , Debelius JW , Thron T , Janssen S , Shastri GG , Ilhan ZE , Challis C , Schretter CE , Rocha S , Gradinaru V , Chesselet M-F , Keshavarzian A , Shannon KM , Krajmalnik-Brown R , Wittung-Stafshede P , Knight R , Mazmanian SK ((2016) ) Gut microbiota regulate motor deficits and neuroinflammation in a model of Parkinson’s disease. Cell 167: , 1469–1480.e12. |
[164] | Matheoud D , Cannon T , Voisin A , Penttinen A-M , Ramet L , Fahmy AM , Ducrot C , Laplante A , Bourque M-J , Zhu L , Cayrol R , Le Campion A , McBride HM , Gruenheid S , Trudeau L-E , Desjardins M ((2019) ) Intestinal infection triggers Parkinson’s disease-like symptoms in Pink1-/- mice. Nature 571: , 565–569. |
[165] | Houser MC , Tansey MG ((2017) ) The gut-brain axis: Is intestinal inflammation a silent driver of Parkinson’s disease pathogenesis? NPJ Parkinsons Dis 3: , 3. |
[166] | Groh KJ , Geueke B , Muncke J ((2017) ) Food contact materials and gut health: Implications for toxicity assessment and relevance of high molecular weight migrants. Food Chem Toxicol 109: , 1–18. |
[167] | Gillois K , Lévêque M , Théodorou V , Robert H , Mercier-Bonin M ((2018) ) Mucus: An underestimated gut target for environmental pollutants and food additives. Microorganisms 6: , 53. |
[168] | Zhang Y , Jin C , Wang X , Shen M , Zhou J , Wu S , Fu Z , Jin Y ((2019) ) Propamocarb exposure decreases the secretion of neurotransmitters and causes behavioral impairments in mice. Environ Toxicol 34: , 22–29. |
[169] | Perez-Pardo P , Dodiya HB , Engen PA , Naqib A , Forsyth CB , Green SJ , Garssen J , Keshavarzian A , Kraneveld AD ((2018) ) Gut bacterial composition in a mouse model of Parkinson’s disease. Benef Microbes 9: , 799–814. |
[170] | Yang X , Qian Y , Xu S , Song Y , Xiao Q ((2017) ) Longitudinal analysis of fecal microbiome and pathologic processes in a rotenone induced mice model of Parkinson’s disease. Front Aging Neurosci 9: , 441. |
[171] | Bhattarai Y , Si J , Pu M , Ross OA , McLean PJ , Till L , Moor W , Grover M , Kandimalla KK , Margolis KG , Farrugia G , Kashyap PC ((2021) ) Role of gut microbiota in regulating gastrointestinal dysfunction and motor symptoms in a mouse model of Parkinson’s disease. Gut Microbes 13: , 1866974. |
[172] | Barichella M , Severgnini M , Cilia R , Cassani E , Bolliri C , Caronni S , Ferri V , Cancello R , Ceccarani C , Faierman S , Pinelli G , De Bellis G , Zecca L , Cereda E , Consolandi C , Pezzoli G ((2019) ) Unraveling gut microbiota in Parkinson’s disease and atypical parkinsonism. Mov Disord 34: , 396–405. |
[173] | Johnson ME , Stringer A , Bobrovskaya L ((2018) ) Rotenone induces gastrointestinal pathology and microbiota alterations in a rat model of Parkinson’s disease. Neurotoxicology 65: , 174–185. |
[174] | Hasegawa S , Goto S , Tsuji H , Okuno T , Asahara T , Nomoto K , Shibata A , Fujisawa Y , Minato T , Okamoto A , Ohno K , Hirayama M ((2015) ) intestinal dysbiosis and lowered serum lipopolysaccharide-binding protein in Parkinson’s disease. PloS One 10: , e0142164. |
[175] | Hill-Burns EM , Debelius JW , Morton JT , Wissemann WT , Lewis MR , Wallen ZD , Peddada SD , Factor SA , Molho E , Zabetian CP , Knight R , Payami H ((2017) ) Parkinson’s disease and Parkinson’s disease medications have distinct signatures of the gut microbiome. Mov Disord 32: , 739–749. |
[176] | Hopfner F , Künstner A , Müller SH , Künzel S , Zeuner KE , Margraf NG , Deuschl G , Baines JF , Kuhlenbäumer G ((2017) ) Gut microbiota in Parkinson disease in a northern German cohort. Brain Res 1667: , 41–45. |
[177] | Scheperjans F , Aho V , Pereira PAB , Koskinen K , Paulin L , Pekkonen E , Haapaniemi E , Kaakkola S , Eerola-Rautio J , Pohja M , Kinnunen E , Murros K , Auvinen P ((2015) ) Gut microbiota are related to Parkinson’s disease and clinical phenotype. Mov Disord 30: , 350–358. |
[178] | Bedarf JR , Hildebrand F , Coelho LP , Sunagawa S , Bahram M , Goeser F , Bork P , Wüllner U ((2017) ) Functional implications of microbial and viral gut metagenome changes in early stage L-DOPA-naïve Parkinson’s disease patients. Genome Med 9: , 39. |
[179] | Keshavarzian A , Green SJ , Engen PA , Voigt RM , Naqib A , Forsyth CB , Mutlu E , Shannon KM ((2015) ) Colonic bacterial composition in Parkinson’s disease. Mov Disord 30: , 1351–1360. |
[180] | Queipo-Ortuño MI , Seoane LM , Murri M , Pardo M , Gomez-Zumaquero JM , Cardona F , Casanueva F , Tinahones FJ ((2013) ) Gut microbiota composition in male rat models under different nutritional status and physical activity and its association with serum leptin and ghrelin levels. PloS One 8: , e65465. |
[181] | Bayliss JA , Lemus MB , Stark R , Santos VV , Thompson A , Rees DJ , Galic S , Elsworth JD , Kemp BE , Davies JS , Andrews ZB ((2016) ) Ghrelin-AMPK signaling mediates the neuroprotective effects of calorie restriction in Parkinson’s disease. J Neurosci 36: , 3049–3063. |
[182] | Wang K , Zhang C , Zhang B , Li G , Shi G , Cai Q , Huang M ((2022) ) Gut dysfunction may be the source of pathological aggregation of alpha-synuclein in the central nervous system through Paraquat exposure in mice. Ecotoxicol Environ Saf 246: , 114152. |
[183] | Li Y , Zuo Z , Zhang B , Luo H , Song B , Zhou Z , Chang X ((2022) ) Impacts of early-life paraquat exposure on gut microbiota and body weight in adult mice. Chemosphere 291: , 133135. |
[184] | Souza da APS , Barros WMA , Silva JML , Silva MRM , Silva ABJ , Fernandes de MSS , dos Santos MERA , da Silva ML , do Carmo TS , Silva RKP , da Silva KG , de Souza SL , Souza de VON ((2021) ) Effect of metabolic syndrome on Parkinson’s disease: A systematic review. Clinics 76: , e3379. |
[185] | Schönbrunn E , Eschenburg S , Shuttleworth WA , Schloss JV , Amrhein N , Evans JNS , Kabsch W ((2001) ) Interaction of the herbicide glyphosate with its target enzyme 5-enolpyruvylshikimate 3-phosphate synthase in atomic detail. Proc Natl Acad Sci U S A 98: , 1376–1380. |
[186] | Leino L , Tall T , Helander M , Saloniemi I , Saikkonen K , Ruuskanen S , Puigbò P ((2021) ) Classification of the glyphosate target enzyme (5-enolpyruvylshikimate-3-phosphate synthase) for assessing sensitivity of organisms to the herbicide. J Hazard Mater 408: , 124556. |
[187] | Mesnage R , Teixeira M , Mandrioli D , Falcioni L , Ducarmon QR , Zwittink RD , Mazzacuva F , Caldwell A , Halket J , Amiel C , Panoff J-M , Belpoggi F , Antoniou MN ((2021) ) Use of shotgun metagenomics and metabolomics to evaluate the impact of glyphosate or roundup MON 52276 on the gut microbiota and serum metabolome of Sprague-Dawley rats. Environ Health Perspect 129: , 017005. |
[188] | Aitbali Y , Ba-M’hamed S , Elhidar N , Nafis A , Soraa N , Bennis M ((2018) ) Glyphosate based- herbicide exposure affects gut microbiota, anxiety and depression-like behaviors in mice. Neurotoxicol Teratol 67: , 44–49. |
[189] | Lozano VL , Defarge N , Rocque L-M , Mesnage R , Hennequin D , Cassier R , de Vendômois JS , Panoff J-M , Séralini G-E , Amiel C ((2018) ) Sex-dependent impact of Roundup on the rat gut microbiome. Toxicol Rep 5: , 96–107. |
[190] | Mao Q , Manservisi F , Panzacchi S , Mandrioli D , Menghetti I , Vornoli A , Bua L , Falcioni L , Lesseur C , Chen J , Belpoggi F , Hu J ((2018) ) The Ramazzini Institute 13-week pilot study on glyphosate and Roundup administered at human-equivalent dose to Sprague Dawley rats: Effects on the microbiome. Environ Health 17: , 50. |
[191] | Motta EVS , Raymann K , Moran NA ((2018) ) Glyphosate perturbs the gut microbiota of honey bees. Proc Natl Acad Sci U S A 115: , 10305–10310. |
[192] | Shehata AA , Schrödl W , Aldin Alaa A , Hafez HM , Krüger M ((2013) ) The effect of glyphosate on potential pathogens and beneficial members of poultry microbiota in vitro. Curr Microbiol 66: , 350–358. |
[193] | Ackermann W , Coenen M , Schrödl W , Shehata AA , Krüger M ((2015) ) The influence of glyphosate on the microbiota and production of botulinum neurotoxin during ruminal fermentation. Curr Microbiol 70: , 374–382. |
[194] | Riede S , Toboldt A , Breves G , Metzner M , Köhler B , Bräunig J , Schafft H , Lahrssen-Wiederholt M , Niemann L ((2016) ) Investigations on the possible impact of a glyphosate-containing herbicide on ruminal metabolism and bacteria in vitro by means of the ‘Rumen Simulation Technique.’ . J Appl Microbiol 121: , 644–656. |
[195] | Krüger M , Shehata AA , Schrödl W , Rodloff A ((2013) ) Glyphosate suppresses the antagonistic effect of Enterococcus spp. on Clostridium botulinum. Anaerobe 20: , 74–78. |
[196] | You M-J , Shin G-W , Lee C-S ((2015) ) Clostridium tertium bacteremia in a patient with glyphosate ingestion. Am J Case Rep 16: , 4–7. |
[197] | Mesnage R , Antoniou MN ((2020) ) Computational modelling provides insight into the effects of glyphosate on the shikimate pathway in the human gut microbiome. Curr Res Toxicol 1: , 25–33. |
[198] | Samsel A , Seneff S ((2013) ) Glyphosate’s suppression of cytochrome P450 enzymes and amino acid biosynthesis by the gut microbiome: Pathways to modern diseases. Entropy 15: , 1416–1463. |
[199] | Sampson TR , Mazmanian SK ((2015) ) Control of brain development, function, and behavior by the microbiome. Cell Host Microbe 17: , 565–576. |
[200] | Fukumoto S , Tatewaki M , Yamada T , Fujimiya M , Mantyh C , Voss M , Eubanks S , Harris M , Pappas TN , Takahashi T ((2003) ) Short-chain fatty acids stimulate colonic transit via intraluminal 5-HT release in rats. Am J Physiol-Regul Integr Comp Physiol 284: , R1269–R1276. |
[201] | Yano JM , Yu K , Donaldson GP , Shastri GG , Ann P , Ma L , Nagler CR , Ismagilov RF , Mazmanian SK , Hsiao EY ((2015) ) Indigenous bacteria from the gut microbiota regulate host serotonin biosynthesis. Cell 161: , 264–276. |
[202] | O’Mahony SM , Marchesi JR , Scully P , Codling C , Ceolho A-M , Quigley EMM , Cryan JF , Dinan TG ((2009) ) Early life stress alters behavior, immunity, and microbiota in rats: Implications for irritable bowel syndrome and psychiatric illnesses. Biol Psychiatry 65: , 263–267. |
[203] | Pu Y , Yang J , Chang L , Qu Y , Wang S , Zhang K , Xiong Z , Zhang J , Tan Y , Wang X , Fujita Y , Ishima T , Wan D , Hwang SH , Hammock BD , Hashimoto K ((2020) ) Maternal glyphosate exposure causes autism-like behaviors in offspring through increased expression of soluble epoxide hydrolase. Proc Natl Acad Sci U S A 117: , 11753–11759. |
[204] | Halliday GM , Leverenz JB , Schneider JS , Adler CH ((2014) ) The neurobiological basis of cognitive impairment in Parkinson’s disease. Mov Disord 29: , 634–650. |
[205] | Chikatimalla R , Dasaradhan T , Koneti J , Cherukuri SP , Kalluru R , Gadde S ((2022) ) Depression in Parkinson’s disease: A narrative review. Cureus 14: , e27750. |
[206] | Perl DP , Olanow CW ((2007) ) The neuropathology of manganese-induced Parkinsonism. J Neuropathol Exp Neurol 66: , 675–682. |
[207] | Olanow CW ((2004) ) Manganese-induced parkinsonism and Parkinson’s disease. Ann N Y Acad Sci 1012: , 209–223. |
[208] | Lucchini RG , Martin CJ , Doney BC ((2009) ) From manganism to manganese-induced parkinsonism: A conceptual model based on the evolution of exposure. Neuromolecular Med 11: , 311–321. |
[209] | Levy BS , Nassetta WJ ((2003) ) Neurologic effects of manganese in humans: A review. Int J Occup Environ Health 9: , 153–163. |
[210] | Willis AW , Evanoff BA , Lian M , Galarza A , Wegrzyn A , Schootman M , Racette BA ((2010) ) Metal emissions and urban incident Parkinson disease: A community health study of Medicare beneficiaries by using geographic information systems. Am J Epidemiol 172: , 1357–1363. |
[211] | Samsel A , Seneff S ((2015) ) Glyphosate, pathways to modern diseases III: Manganese, neurological diseases, and associated pathologies. Surg Neurol Int 6: , 45. |
[212] | Archibald FS , Fridovich I ((1981) ) Manganese, superoxide dismutase, and oxygen tolerance in some lactic acid bacteria. J Bacteriol 146: , 928–936. |
[213] | Terkelsen MH , Hvingelby VS , Pavese N ((2022) ) Molecular imaging of the GABAergic system in Parkinson’s disease and atypical parkinsonisms. Curr Neurol Neurosci Rep 22: , 867–879. |
[214] | Tette F-M , Kwofie SK , Wilson MD ((2022) ) Therapeutic anti-depressant potential of microbial GABA produced by Lactobacillus rhamnosus strains for GABAergic signaling restoration and inhibition of addiction-induced HPA axis hyperactivity. Curr Issues Mol Biol 44: , 1434–1451. |
[215] | Finley JW , Davis CD ((1999) ) Manganese deficiency and toxicity: Are high or low dietary amounts of manganese cause for concern? Biofactors 10: , 15–24. |
[216] | Samsel A , Seneff S ((2013) ) Glyphosate, pathways to modern diseases II: Celiac sprue and gluten intolerance. Interdiscip Toxicol 6: , 159–184. |
[217] | Ke T , Sidoryk-Wegrzynowicz M , Pajarillo E , Rizor A , Soares FAA , Lee E , Aschner M ((2019) ) Role of astrocytes in manganese neurotoxicity revisited. Neurochem Res 44: , 2449–2459. |
[218] | Lindquist NG , Larsson BS , Lydén-Sokolowski A ((1987) ) Neuromelanin and its possible protective and destructive properties. Pigment Cell Res 1: , 133–136. |
[219] | Faria MA ((2015) ) Glyphosate, neurological diseases –and the scientific method. Surg Neurol Int 6: , 132. |
[220] | Peillex C , Pelletier M ((2020) ) The impact and toxicity of glyphosate and glyphosate-based herbicides on health and immunity. J Immunotoxicol 17: , 163–174. |
[221] | Zhu Y , Huan F , Wang J , Xie X , Yu G , Wang X , Jiang L , Gao R , Xiao H , Ding H , Wang J ((2020) ) 1-Methyl-4-phenyl-1,2,3,6-tetrahydropyridine induced Parkinson’s disease in mouse: Potential association between neurotransmitter disturbance and gut microbiota dysbiosis. ACS Chem Neurosci 11: , 3366–3376. |
[222] | Lai F , Jiang R , Xie W , Liu X , Tang Y , Xiao H , Gao J , Jia Y , Bai Q ((2018) ) Intestinal pathology and gut microbiota alterations in a methyl-4-phenyl-1,2,3,6-tetrahydropyridine (MPTP) mouse model of Parkinson’s disease. Neurochem Res 43: , 1986–1999. |
[223] | Xie W , Gao J , Jiang R , Liu X , Lai F , Tang Y , Xiao H , Jia Y , Bai Q ((2020) ) Twice subacute MPTP administrations induced time-dependent dopaminergic neurodegeneration and inflammation in midbrain and ileum, as well as gut microbiota disorders in PD mice. Neurotoxicology 76: , 200–212. |
[224] | Sun M-F , Zhu Y-L , Zhou Z-L , Jia X-B , Xu Y-D , Yang Q , Cui C , Shen Y-Q ((2018) ) Neuroprotective effects of fecal microbiota transplantation on MPTP-induced Parkinson’s disease mice: Gut microbiota, glial reaction and TLR4/TNF-α signaling pathway. Brain Behav Immun 70: , 48–60. |
[225] | Unger MM , Spiegel J , Dillmann K-U , Grundmann D , Philippeit H , Bürmann J , Faßbender K , Schwiertz A , Schäfer K-H ((2016) ) Short chain fatty acids and gut microbiota differ between patients with Parkinson’s disease and age-matched controls. Parkinsonism Relat Disord 32: , 66–72. |
[226] | Joly Condette C , Bach V , Mayeur C , Gay-Quéheillard J , Khorsi-Cauet H ((2015) ) Chlorpyrifos exposure during perinatal period affects intestinal microbiota associated with delay of maturation of digestive tract in rats. J Pediatr Gastroenterol Nutr 61: , 30–40. |
[227] | Nasuti C , Coman MM , Olek RA , Fiorini D , Verdenelli MC , Cecchini C , Silvi S , Fedeli D , Gabbianelli R ((2016) ) Changes on fecal microbiota in rats exposed to permethrin during postnatal development. Environ Sci Pollut Res 23: , 10930–10937. |
[228] | Reygner J , Joly Condette C , Bruneau A , Delanaud S , Rhazi L , Depeint F , Abdennebi-Najar L , Bach V , Mayeur C , Khorsi-Cauet H ((2016) ) Changes in composition and function of human intestinal microbiota exposed to chlorpyrifos in oil as assessed by the SHIME® model. Int J Environ Res Public Health 13: , 1088. |
[229] | Iszatt N , Janssen S , Lenters V , Dahl C , Stigum H , Knight R , Mandal S , Peddada S , González A , Midtvedt T , Eggesbø M ((2019) ) Environmental toxicants in breast milk of Norwegian mothers and gut bacteria composition and metabolites in their infants at 1 month. Microbiome 7: , 34. |
[230] | Caricilli AM , Castoldi A , Câmara NOS ((2014) ) Intestinal barrier: A gentlemen’s agreement between microbiota and immunity. World J Gastrointest Pathophysiol 5: , 18–32. |
[231] | Ivanov II , Honda K ((2012) ) Intestinal commensal microbes as immune modulators. Cell Host Microbe 12: , 496–508. |
[232] | Nishio J , Honda K ((2012) ) Immunoregulation by the gut microbiota. Cell Mol Life Sci 69: , 3635–3650. |
[233] | Stanaway IB , Wallace JC , Shojaie A , Griffith WC , Hong S , Wilder CS , Green FH , Tsai J , Knight M , Workman T , Vigoren EM , McLean JS , Thompson B , Faustman EM ((2016) ) Human oral buccal microbiomes are associated with farmworker status and azinphos-methyl agricultural pesticide exposure. Appl Environ Microbiol 83: , e02149–16. |
[234] | Heintz-Buschart A , Pandey U , Wicke T , Sixel-Döring F , Janzen A , Sittig-Wiegand E , Trenkwalder C , Oertel WH , Mollenhauer B , Wilmes P ((2018) ) The nasal and gut microbiome in Parkinson’s disease and idiopathic rapid eye movement sleep behavior disorder. Mov Disord 33: , 88–98. |
[235] | Walter J , Armet AM , Finlay BB , Shanahan F ((2020) ) Establishing or exaggerating causality for the gut microbiome: Lessons from human microbiota-associated rodents. Cell 180: , 221–232. |
[236] | Pu Y , Chang L , Qu Y , Wang S , Zhang K , Hashimoto K ((2019) ) Antibiotic-induced microbiome depletion protects against MPTP-induced dopaminergic neurotoxicity in the brain. Aging 11: , 6915–6929. |
[237] | Huang R ((2021) ) Gut microbiota: A key regulator in the effects of environmental hazards on modulates insulin resistance. Front Cell Infect Microbiol 11: , 800432. |
[238] | Hirayama M , Ohno K ((2021) ) Parkinson’s disease and gut microbiota. Ann Nutr Metab 77 Suppl 2: , 28–35. |
[239] | Roe K ((2022) ) An alternative explanation for Alzheimer’s disease and Parkinson’s disease initiation from specific antibiotics, gut microbiota dysbiosis and neurotoxins. Neurochem Res 47: , 517–530. |
[240] | Croom E (2012) Chapter Three –Metabolism of xenobiotics of human environments. In Progress in Molecular Biology and Translational Science, Hodgson E, ed. Academic Press, pp. 31–88. |
[241] | Collins SL , Patterson AD ((2020) ) The gut microbiome: An orchestrator of xenobiotic metabolism. Acta Pharm Sin B 10: , 19–32. |
[242] | Nakov R , Velikova T ((2020) ) Chemical metabolism of xenobiotics by gut microbiota. Curr Drug Metab 21: , 260–269. |
[243] | Meng Z , Liu L , Yan S , Sun W , Jia M , Tian S , Huang S , Zhou Z , Zhu W ((2020) ) Gut microbiota: A key factor in the host health effects induced by pesticide exposure? J Agric Food Chem 68: , 10517–10531. |
[244] | Cardoso S , Moreira PI ((2020) ) Antidiabetic drugs for Alzheimer’s and Parkinson’s diseases: Repurposing insulin, metformin, and thiazolidinediones. Int Rev Neurobiol 155: , 37–64. |
[245] | Unger MM , Möller JC , Mankel K , Eggert KM , Bohne K , Bodden M , Stiasny-Kolster K , Kann PH , Mayer G , Tebbe JJ , Oertel WH ((2011) ) Postprandial ghrelin response is reduced in patients with Parkinson’s disease and idiopathic REM sleep behaviour disorder: A peripheral biomarker for early Parkinson’s disease? J Neurol 258: , 982–990. |
[246] | Outeiro TF , Alcalay RN , Antonini A , Attems J , Bonifati V , Cardoso F , Chesselet M-F , Hardy J , Madeo G , McKeith I , Mollenhauer B , Moore DJ , Rascol O , Schlossmacher MG , Soreq H , Stefanis L , Ferreira JJ ((2023) ) Defining the riddle in order to solve it: There is more than one “Parkinson’s disease.” . Mov Disord 38: , 1127–1142. |
[247] | Horsager J , Knudsen K , Sommerauer M ((2022) ) Clinical and imaging evidence of brain-first and body-first Parkinson’s disease. Neurobiol Dis 164: , 105626. |
[248] | Horsager J , Andersen KB , Knudsen K , Skjærbæk C , Fedorova TD , Okkels N , Schaeffer E , Bonkat SK , Geday J , Otto M , Sommerauer M , Danielsen EH , Bech E , Kraft J , Munk OL , Hansen SD , Pavese N , Göder R , Brooks DJ , Berg D , Borghammer P ((2020) ) Brain-first versus body-first Parkinson’s disease: A multimodal imaging case-control study. Brain 143: , 3077–3088. |
[249] | Merola A , Romagnolo A , Dwivedi AK , Padovani A , Berg D , Garcia-Ruiz PJ , Fabbri M , Artusi CA , Zibetti M , Lopiano L , Pilotto A , Bonacina S , Morgante F , Zeuner K , Griewing C , Schaeffer E , Rodriguez-Porcel F , Kauffman M , Turcano P , de Oliveira LM , Palermo G , Shanks E , Del Sorbo F , Bonvegna S , Savica R , Munhoz RP , Ceravolo R , Cilia R , Espay AJ ((2020) ) Benign versus malignant Parkinson disease: The unexpected silver lining of motor complications. J Neurol 267: , 2949–2960. |
[250] | Nuzum ND , Loughman A , Szymlek-Gay EA , Hendy A , Teo W-P , Macpherson H ((2020) ) Gut microbiota differences between healthy older adults and individuals with Parkinson’s disease: A systematic review. Neurosci Biobehav Rev 112: , 227–241. |
[251] | Nuzum ND , Loughman A , Szymlek-Gay EA , Teo W-P , Hendy AM , Macpherson H ((2022) ) To the gut microbiome and beyond: The brain-first or body-first hypothesis in Parkinson’s disease. Front Microbiol 13: , 791213. |
[252] | Raimondi S , Musmeci E , Candeliere F , Amaretti A , Rossi M ((2021) ) Identification of mucin degraders of the human gut microbiota. Sci Rep 11: , 11094. |
[253] | Sandor C , Robertson P , Lang C , Heger A , Booth H , Vowles J , Witty L , Bowden R , Hu M , Cowley SA , Wade-Martins R , Webber C ((2017) ) Transcriptomic profiling of purified patient-derived dopamine neurons identifies convergent perturbations and therapeutics for Parkinson’s disease. Hum Mol Genet 26: , 552–566. |
[254] | Di Maio R , Hoffman EK , Rocha EM , Keeney MT , Sanders LH , De Miranda BR , Zharikov A , Van Laar A , Stepan AF , Lanz TA , Kofler JK , Burton EA , Alessi DR , Hastings TG , Greenamyre JT ((2018) ) LRRK2 activation in idiopathic Parkinson’s disease. Sci Transl Med 10: , eaar5429. |
[255] | Rocha EM , De Miranda BR , Castro S , Drolet R , Hatcher NG , Yao L , Smith SM , Keeney MT , Di Maio R , Kofler J , Hastings TG , Greenamyre JT ((2020) ) LRRK2 inhibition prevents endolysosomal deficits seen in human Parkinson’s disease. Neurobiol Dis 134: , 104626. |
[256] | Dwyer Z , Rudyk C , Thompson A , Farmer K , Fenner B , Fortin T , Derksen A , Sun H , Hayley S , CLINT (Canadian LRRK2 in inflammation team) ((2020) ) Leucine-rich repeat kinase-2 (LRRK2) modulates microglial phenotype and dopaminergic neurodegeneration. Neurobiol Aging 91: , 45–55. |
[257] | Rudyk C , Dwyer Z , Hayley S , CLINT membership ((2019) ) Leucine-rich repeat kinase-2 (LRRK2) modulates paraquat-induced inflammatory sickness and stress phenotype. J Neuroinflammation 16: , 120. |
[258] | De Miranda BR , Castro SL , Rocha EM , Bodle CR , Johnson KE , Greenamyre JT ((2021) ) The industrial solvent trichloroethylene induces LRRK2 kinase activity and dopaminergic neurodegeneration in a rat model of Parkinson’s disease. Neurobiol Dis 153: , 105312. |
[259] | Chen J , Su P , Luo W , Chen J ((2018) ) Role of LRRK2 in manganese-induced neuroinflammation and microglial autophagy. Biochem Biophys Res Commun 498: , 171–177. |
[260] | Kim J , Pajarillo E , Rizor A , Son D-S , Lee J , Aschner M , Lee E ((2019) ) LRRK2 kinase plays a critical role in manganese-induced inflammation and apoptosis in microglia. PloS One 14: , e0210248. |
[261] | Zhao Y , Dzamko N ((2019) ) Recent developments in LRRK2-targeted therapy for Parkinson’s disease. Drugs 79: , 1037–1051. |
[262] | Pristner M , Warth B ((2020) ) Drug-exposome interactions: The next frontier in precision medicine. Trends Pharmacol Sci 41: , 994–1005. |
[263] | Kannarkat GT , Cook DA , Lee J-K , Chang J , Chung J , Sandy E , Paul KC , Ritz B , Bronstein J , Factor SA , Boss JM , Tansey MG ((2015) ) Common genetic variant association with altered HLA expression, synergy with pyrethroid exposure, and risk for Parkinson’s disease: An observational and case-control study. NPJ Parkinsons Dis 1: , 15002-. |
[264] | Goldman SM , Kamel F , Ross GW , Bhudhikanok GS , Hoppin JA , Korell M , Marras C , Meng C , Umbach DM , Kasten M , Chade AR , Comyns K , Richards MB , Sandler DP , Blair A , Langston JW , Tanner CM ((2012) ) Genetic modification of the association of paraquat and Parkinson’s disease. Mov Disord 27: , 1652–1658. |
[265] | Rösler TW , Salama M , Shalash AS , Khedr EM , El-Tantawy A , Fawi G , El-Motayam A , El-Seidy E , El-Sherif M , El-Gamal M , Moharram M , El-Kattan M , Abdel-Naby M , Ashour S , Müller U , Dempfle A , Kuhlenbäumer G , Höglinger GU ((2018) ) K-variant BCHE and pesticide exposure: Gene-environment interactions in a case–control study of Parkinson’s disease in Egypt. Sci Rep 8: , 16525. |
[266] | Nandar W , Connor JR ((2011) ) HFE gene variants affect iron in the brain. J Nutr 141: , 729S–739S. |
[267] | Nixon AM , Meadowcroft MD , Neely EB , Snyder AM , Purnell CJ , Wright J , Lamendella R , Nandar W , Huang X , Connor JR ((2018) ) HFE genotype restricts the response to paraquat in a mouse model of neurotoxicity. J Neurochem 145: , 299–311. |
[268] | Dorsey ER , Bloem BR ((2018) ) The Parkinson pandemic-a call to action. JAMA Neurol 75: , 9–10. |
[269] | Gama J , Neves B , Pereira A ((2022) ) Chronic effects of dietary pesticides on the gut microbiome and neurodevelopment. Front Microbiol 13: , 931440. |
[270] | Baranski R , Klimek-Chodacka M , Lukasiewicz A ((2019) ) Approved genetically modified (GM) horticultural plants: A 25-year perspective. Folia Hortic 31: , 3–49. |
[271] | Arif I , Batool M , Schenk PM ((2020) ) Plant microbiome engineering: Expected benefits for improved crop growth and resilience. Trends Biotechnol 38: , 1385–1396. |
[272] | Fletcher SJ , Reeves PT , Hoang BT , Mitter N ((2020) ) A perspective on RNAi-based biopesticides. Front Plant Sci 11: , 51. |
[273] | Sarkar S , Gil JDB , Keeley J , Jansen K (2021) The use of pesticides in developing countries and their impact on health and the right to food, European Union, Brussels. |
[274] | Darweesh SKL , Vermeulen R , Bloem BR , Peters S ((2022) ) Exposure to pesticides predicts prodromal feature of Parkinson’s disease: Public health implications. Mov Disord 37: , 883–885. |
[275] | Logroscino G ((2005) ) The role of early life environmental risk factors in Parkinson disease: What is the evidence? Environ Health Perspect 113: , 1234–1238. |
[276] | Furlong M , Tanner CM , Goldman SM , Bhudhikanok GS , Blair A , Chade A , Comyns K , Hoppin JA , Kasten M , Korell M , Langston JW , Marras C , Meng C , Richards M , Ross GW , Umbach DM , Sandler DP , Kamel F ((2015) ) Protective glove use and hygiene habits modify the associations of specific pesticides with Parkinson’s disease. Environ Int 75: , 144–150. |
[277] | Shrestha S , Parks CG , Umbach DM , Richards-Barber M , Hofmann JN , Chen H , Blair A , Beane Freeman LE , Sandler DP ((2020) ) Pesticide use and incident Parkinson’s disease in a cohort of farmers and their spouses. Environ Res 191: , 110186. |
[278] | Cherukuri H , Pramoda K , Rohini D , Thunga G , Vijaynarayana K , Sreedharan N , Varma M , Pandit V ((2014) ) Demographics, clinical characteristics and management of herbicide poisoning in tertiary care hospital. Toxicol Int 21: , 209–213. |
[279] | Kamel F , Goldman SM , Umbach DM , Chen H , Richardson G , Barber MR , Meng C , Marras C , Korell M , Kasten M , Hoppin JA , Comyns K , Chade A , Blair A , Bhudhikanok GS , Webster Ross G , William Langston J , Sandler DP , Tanner CM ((2014) ) Dietary fat intake, pesticide use, and Parkinson’s disease. Parkinsonism Relat Disord 20: , 82–87. |
[280] | Thangaleela S , Sivamaruthi BS , Kesika P , Bharathi M , Chaiyasut C ((2022) ) Role of the gut-brain axis, gut microbial composition, diet, and probiotic intervention in Parkinson’s disease. Microorganisms 10: , 1544. |
[281] | Zacharias HU , Kaleta C , Cossais F , Schaeffer E , Berndt H , Best L , Dost T , Glüsing S , Groussin M , Poyet M , Heinzel S , Bang C , Siebert L , Demetrowitsch T , Leypoldt F , Adelung R , Bartsch T , Bosy-Westphal A , Schwarz K , Berg D ((2022) ) Microbiome and metabolome insights into the role of the gastrointestinal–brain axis in Parkinson’s and Alzheimer’s disease: Unveiling potential therapeutic targets. Metabolites 12: , 1222. |
[282] | Breckenridge CB , Berry C , Chang ET , Sielken RL Jr. , Mandel JS ((2016) ) Association between Parkinson’s disease and cigarette smoking, rural living, well-water consumption, farming and pesticide use: Systematic review and meta-analysis. PLoS One 11: , e0151841. |
[283] | Murata H , Barnhill LM , Bronstein JM ((2022) ) Air pollution and the risk of Parkinson’s disease: A review. Mov Disord 37: , 894–904. |
[284] | Lee P-C , Bordelon Y , Bronstein J , Ritz B ((2012) ) Traumatic brain injury, paraquat exposure, and their relationship to Parkinson disease. Neurology 79: , 2061–2066. |
[285] | Goldman SM , Kamel F , Ross GW , Jewell SA , Bhudhikanok GS , Umbach D , Marras C , Hauser RA , Jankovic J , Factor SA , Bressman S , Lyons KE , Meng C , Korell M , Roucoux DF , Hoppin JA , Sandler DP , Langston JW , Tanner CM ((2012) ) Head injury, α-synuclein Rep1, and Parkinson’s disease. Ann Neurol 71: , 40–48. |