Dynamic filtration in baleen whales: recent discoveries and emerging trends
- 1Department of Biology, Hampden-Sydney College, Hampden-Sydney, VA, United States
- 2Department of Physics and WATER Institute, Saint Louis University, St. Louis, MO, United States
Recent findings have greatly improved our understanding of mysticete oral filtration, and have upended the traditional view of baleen filtration as a simple process. Flow tank experiments, telemetric tag deployment on whales, and other lab and field methods continue to yield new data and ideas. These suggest that several mechanisms arose from ecological, morphological, and biomechanical adaptations facilitating the evolution of extreme body size in Mysticeti. Multiple lines of evidence strongly support a characterization of baleen filtration as a conceptually dynamic process, varying according to diverse intraoral locations and times of the filtration process, and to other prevailing conditions. We review and highlight these lines of evidence as follows. First, baleen appears to work as a complex metafilter comprising multiple components with differing properties. These include major and minor plates and eroded fringes (AKA bristles or hairs), as well as whole baleen racks. Second, it is clear that different whale species rely on varied ecological filtration modes ranging from slow skimming to high-speed lunging, with other possibilities in between. Third, baleen filtration appears to be a highly dynamic and flow-dependent process, with baleen porosity not only varying across sites within a single rack, but also by flow direction, speed, and volume. Fourth, findings indicate that baleen (particularly of balaenid whales and possibly other species) generally functions not as a simple throughput sieve, but instead likely uses cross-flow or other tangential filtration, as in many biological systems. Fifth, evidence reveals that the time course of baleen filtration, including rate of filter filling and clearing, appears to be more complex than formerly envisioned. Flow direction, and possibly plate and fringe orientation, appears to change during different stages of ram filtration and water expulsion. Sixth, baleen’s flexibility and related biomechanical properties varies by location within the whole filter (=rack), leading to varying filtration conditions and outcomes. Seventh, the means of clearing/cleaning the baleen filter, whether by hydraulic, hydrodynamic, or mechanical methods, appears to vary by species and feeding type, notably intermittent lunging versus continuous skimming. Together, these and other findings of the past two decades have greatly elucidated processes of baleen filtration, and heightened the need for further research. Many aspects of baleen filtration may pertain to other biological filters; designers can apply several aspects to artificial filtration, both to better understand natural systems and to design and manufacture more effective synthetic filters. Understanding common versus unique features of varied filtration phenomena, both biological and artificial, will continue to aid scientific and technical understanding, enable fruitful interdisciplinary partnerships, and yield new filter designs.
1 Introduction
Until recently, accounts of filter feeding in baleen whales (Cetacea: Mysticeti) have been based almost solely on speculative inference from classical anatomy and ecology. These accounts were constructed mainly from limited and brief ship-board observations of whale foraging, and from beachside necropsy dissections of stranded whales, often in poor physical condition. Thanks to the dedicated efforts of many researchers investigating and speculating over centuries, scientists were able to piece together broad outlines of whale feeding, such as the fundamental distinction between steady-state skimming versus brief lunge gulping in various mysticete taxa (Slijper, 1962; Nemoto, 1970; Berta et al., 2015). However, until recently, overall accounts of whale feeding, even in leading publications such as the peer-reviewed and edited Encyclopedia of Marine Mammals (EMM), offered only vague description of the processes of baleen filtration. For example, here are four entries from the second (2008) EMM edition:
“Periodically the mouth is closed and plankton are removed from the baleen by the tongue, and ingested.” (EMM entry on “Feeding Morphology”; Marshall, 2008)
“Baleen whales … force water containing food out through baleen plates, and then transfer trapped food back to the gullet. The tongue is presumed to be involved.” (EMM entry on “Baleen Whales (Mysticetes)”; Bannister, 2008)
“Water is expelled by the pouch and tongue through the still exposed baleen plates. Once the water is expelled, the prey is swallowed.” (EMM entry on “Blue Whales”; Sears and Perrin, 2008)
“The tongue and the elastic properties of the ventral walls of the throat act in concert to force water out through the baleen.” (EMM entry on “Filter Feeding”; Croll et al., 2008)
Citation of these four sources is by no means intended to criticize EMM authors or editors for vague descriptions, but rather to point out that until a mere two decades ago, these were the best explanations the world’s top scientists could offer. In short, our knowledge of whale filtration remained limited, even among experts in the field. This was true not only with the EMM, but also other monographs written by acknowledged experts (e.g., Slijper, 1962; Gaskin, 1982, and the Handbook of Marine Mammal series, whose final volume was published by Ridgway and Harrison, 1999), whereas recent works (e.g., Goldbogen et al., 2017b; Pyenson, 2018; Marshall and Pyenson, 2019) present a far more realistic view of whale feeding.
Fortunately, the past two decades have witnessed an explosive rise in the application of new techniques and technologies, many long used in other fields of science, ushering in a new era for our understanding of mysticete feeding, and particularly of baleen filtration, both in specific details and general outline. Examples of these new techniques and studies notably include but are not limited to hypothesis-based laboratory experiments (e.g., Lambertsen, 1983; Werth, 2013), tag data (Goldbogen et al., 2017a), photogrammetry (e.g., Lambertsen et al., 1989), aerial drones (Werth et al., 2019b), engineering methods (Lambertsen et al., 2005), computational fluid dynamics (Zhu et al., 2020a, Zhu et al., 2020b), morphometrics (Werth et al., 2018a), histology (e.g., Werth et al., 2018b), mathematical modeling (Potvin et al., 2009; Potvin and Werth, 2017), and physical modeling (Werth, 2004).
Nonetheless, for all the insights provided by application of novel approaches, there remain many as-yet unanswered questions. Therefore, the aim here is not to provide an exhaustively comprehensive review of recent projects/publications, methods, and findings, but instead to take stock and reflect on major themes and threads arising from these approaches. A roadmap is hereby offered, surveying the current state of the field, including major advances in current understanding as well as suggestions to guide future researchers in addressing current mysteries concerning baleen filtration.
This review reflects the dawning realization that baleen filtration is considerably more complex than previously presumed by generations of researchers (even the authors, formerly). Moreover, baleen filtration is at heart a dynamic and variable process rather than a static or uniform one. Consider that for a large rorqual such as a 25 m blue whale, a typical lunge feeding event involves engulfment of >80-120 m3 of water, which is then filtered through a ~4 m2 filter in roughly 30 seconds, with peak pressures potentially reaching >800–1000 kPa, the equivalent of 106 N/m2 (Werth, 2013). Further, this filter must retain structural integrity and remain functional for the whale’s entire lifespan (presumably 100 years or more; George et al., 2021). It must filter incredibly capacious volumes of water and hold massive volumes of water-borne schooling prey, at astonishing pressures, without breaking, clogging, or otherwise failing. Truly, this is a leading contender for the world’s most dynamic biomechanical event (Goldbogen, 2010).
As researchers gradually fill in details of this former “black box” phenomenon (as evidenced by the nebulous ambiguity of the above-cited EMM entries), two clear conclusions emerge. First, baleen filtration is a far more complex process than previously presumed. Secondly and relatedly, baleen filtration is generally a highly dynamic process with varying inputs and outputs. As summarized in Table 1, mysticete foraging ecology is itself varied, with three variants—skimming and lunge or suction gulping—corresponding to the three main families of extant baleen whales (Balaenidae, Balaenopteridae, and Eschrichtiidae, respectively; Slijper, 1962; Pivorunas, 1979; Werth, 2000; Goldbogen et al., 2017b; Savoca et al., 2021). Nonetheless, mysticete filtration processes are both varying and variable (Werth et al., 2018a; Werth, 2019). Specifically, each baleen filter appears to display varying properties (e.g., porosity) from one filter region or element to another, and these properties also appear to be variable over time as determined by such input parameters as flow speed, direction, and volume. In brief, it is difficult to precisely characterize baleen filtration without specifying detailed operating conditions or attaching qualifying caveats (Werth, 2013).
In the sections that follow, major findings of the past two decades of research on baleen filtration are summarized. We acknowledge that certain variabilities and complexities outlined here are simpler and currently better known than other items, but all alike contribute to the complete picture of baleen filtration, undoubtedly along with numerous other factors whose contributions have yet to be learned or appreciated.
2 Baleen as metafilter with structural complexity & variability
Biological filtration operates on numerous levels and with many functions, including retention of desired filtered material for nutritive gain or elimination of undesired filtered material as excreted waste (Werth, 2019). Baleen does not perform both functions; it solely collects and retains desired material (food) while separating it from seawater. Because of this broad range of function, many biological filters exist, ranging from tissues and organs (e.g., kidneys, livers, and sinusoidal capillaries) to entire ecosystems (e.g., wetlands). In the middle of this continuum lie dedicated filter-feeding structures or whole specialized organs. These may exist outside the body, as in the filter-feeding appendages of copepods, barnacles, and other crustaceans, or they may lie within the body, as in the gill rakers of bony fishes and cartilaginous sharks and rays (Cheer et al., 2012; Paig-Tran et al., 2013; Paig-Tran and Summers, 2014; Wegner, 2015; Divi et al., 2018). Such filter-feeding configurations may be modified from preexisting structures, as in fish gill rakers, the spined tongues of filter-feeding waterfowl, or the complexly cusped dentition of filter-feeding seals, especially crabeater seals, Lobodon carcinophagus, and leopard seals, Hydrurga leptonyx (Werth, 2000; Marshall and Goldbogen, 2015; Hocking et al., 2017b; Marshall and Pyenson, 2019; Hamann and Blanke, 2022). Alternatively, filters may be novel arrangements that evolved de novo, as is the case for baleen (Werth, 2017). This keratinous filtering material, suspended from the palate of all extant species of crown mysticetes, is a neomorphism that evolved roughly 25 million years ago from early, toothed members of stem Mysticeti (Fordyce and Barnes, 1994; Thewissen, 1998; Thewissen and Bajpai, 2001; Thewissen et al., 2009; Uhen, 2010; Marx and Fordyce, 2015; Pyenson and Vermeij, 2016; Marx et al., 2016a, Marx et al., 2016b; Pyenson, 2017; Slater et al., 2017). Although fossilized baleen exists (Esperante et al., 2008; Bisconti, 2012; Gioncada et al., 2016; Collareta et al., 2017; Marx et al., 2017; Bosio et al., 2021), abundant fossil skulls and teeth of early mysticetes reveal that dentition gradually declined in tooth size and number as it was replaced by this new and highly adaptive key innovation (Fordyce, 1980; Fitzgerald, 2010; Berta et al., 2016; Marx et al., 2016a; Geisler et al., 2017; Lambert et al., 2017; Peredo et al., 2017; Hocking et al., 2017a; Fordyce and Marx, 2018; Ekdale and Deméré, 2021; Gatesy et al., 2022). Although baleen functions roughly analogously to filtering dentition, it is not homologous to teeth, the keratinous palatal ridges of some mammals, or any other tissue (Gatesy and O’Leary, 2001; Deméré et al., 2008; Fudge et al., 2009; Gatesy et al., 2013; Thewissen et al., 2017). Like other oral filters, baleen both collects and separates prey items from water, in this case by bulk filtration of schooling prey, whether zooplankton (ranging from <1-10 cm) or fish (generally ~10-20 cm in body length).
Baleen can variously yet simultaneously be considered as a tissue, as a structural material, and (collectively) as a filtering unit roughly equivalent to an organ (Werth, 2017). The entire filter consists of paired “racks” of baleen hanging from each side of the upper jaw, with each rack comprising approximately 300 individual plates of baleen tissue, each of which is roughly triangular in shape (Figure 1). Because each rack is therefore a layered, comb-like structure, each individual baleen plate is also sometimes called a lamina (Young, 2012; Loch et al., 2020). The precise number of plates varies by species (ranging from ~160-360 plates per rack), and to a lesser degree by a whale’s overall body size, which in turn depends on age and sex (Werth et al., 2018a). However, virtually all adult mysticetes have about 300 baleen plates suspended from each side of the rostrum. Baleen plate size varies by species, although plates are generally about 3 mm thick (anteroposteriorly), ~10-20 cm wide (mediolaterally) for most of their length, and 20-40 cm wide at their dorsal-most origin where they emerge from and are anchored within palatal gingiva. In most whales, baleen plates are ~50 cm long (dorsoventrally), but plates range from ~35-75 cm in length for most species, with the major exception of bowhead (Balaena mysticetus) and right whales (Eubalaena spp.), both of Family Balaenidae, whose plates can, in exceptionally large specimens, exceed 4 m in length (Werth, 2000). Plate dimensions vary by position along the rack, with the longest plates found near the center of a rack (Werth et al., 2018a, Werth et al., 2020). In addition to major (main) plates, most whales have smaller minor plates running medially along each rack. A major plate can have one or multiple adjacent minor plates, all of which resemble fragments broken from the medial edge of a major plate (Williamson, 1973; Pivorunas, 1976). As their name suggests, minor plates are considerably smaller in size than major plates (Figure 1). Relative to their reduced size, minor plates likely also play a greatly reduced functional role in filtration, although this has not been adequately addressed.
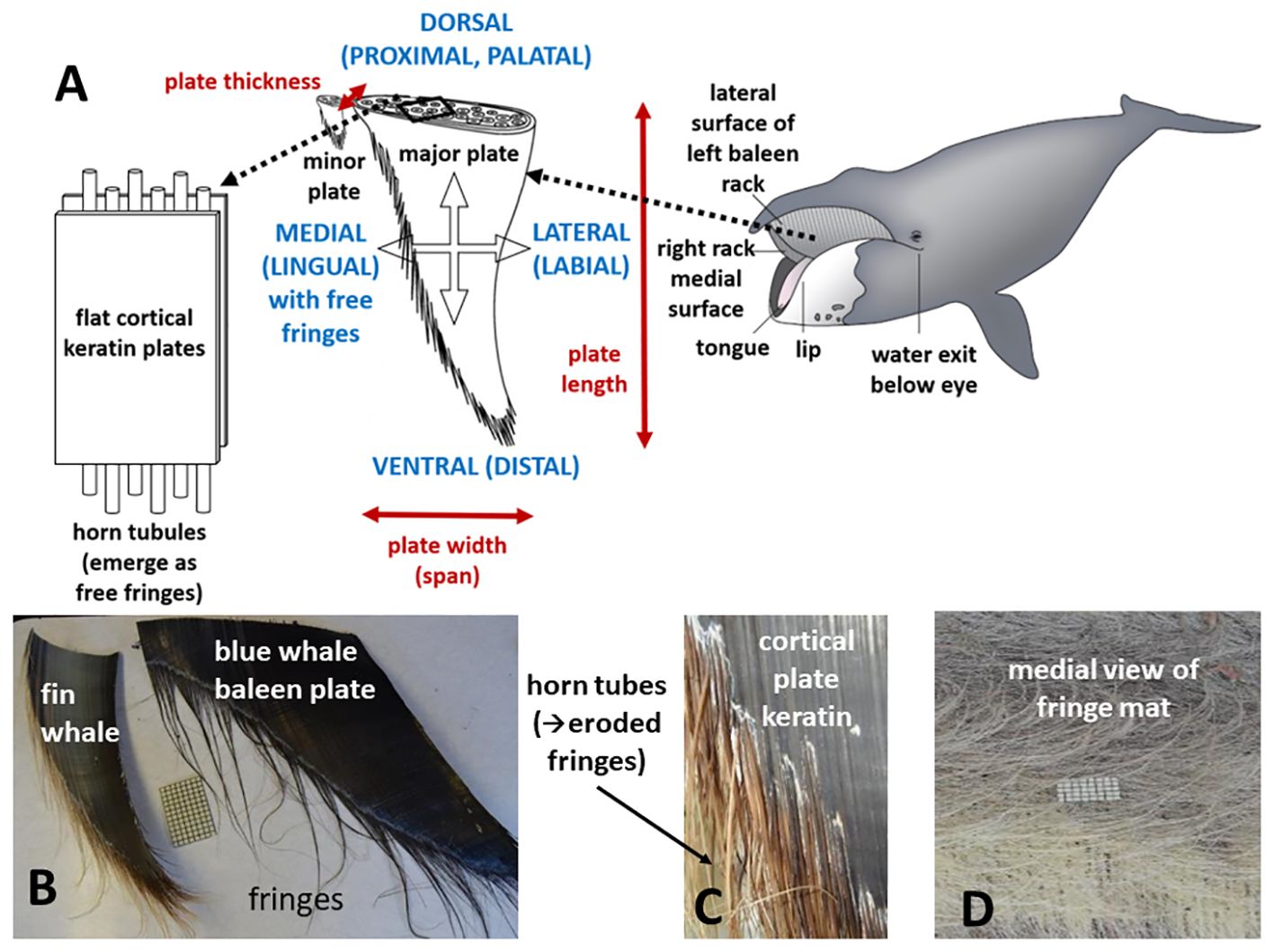
Figure 1 Baleen is a complex oral metafilter comprising different alpha-keratinous elements arranged sandwich-style with flat, fingernail-like plates enclosing hollow hair-like horn tubules (A). Each element has its own filter function along with unique structural and biomechanical properties. Paired racks of baleen tissue (A) each consist of ~300 triangular major plates (B) that erode along their interior (medial, lingual) surfaces (C) to reveal the horn tubes as fringes (bristles; D). An entire rack, major/minor plate, or fringe can separately or collectively serve as a filter element.
Baleen is an ever-growing substance, like many keratinous tissues such as mammalian hair, nails, and claws, but unlike teeth and most other vertebrate filtering materials (Marshall et al., 1991; Wang et al., 2016). In an elegantly simple turnover that balances baleen tissue generation and loss, new filtering material arises to replace eroded material that becomes frayed and shed during filtration (Werth et al., 2020, Werth et al., 2021). This ever-changing nature of filter growth and loss aptly relates baleen’s dynamic structure with its dynamic function. Although baleen growth rates vary somewhat by species and age, in most whales the plates grow about 12-18 cm per year (Sumich, 2001; Werth et al., 2021). Bowhead and right whales, which have much longer plates and thus substantially larger baleen filters, are again the exception; balaenid plates typically grow 20-27 cm per year in adults and can exceed 30 cm per year in juveniles (Werth et al., 2020). In most mysticete species, baleen plates grow disproportionately faster in the first 2-3 years of life. This has been explained as young whales needing to prioritize post-weaning growth of their oral filter to obtain calories needed to sustain growth of other body parts, such that skeletal growth is often delayed or downplayed until the filter attains its full adult proportions (Lubetkin et al., 2008; Fortune et al., 2012; George et al., 2016).
As embryos, mysticetes normally bear multiple transient tooth germs or anlagen that do not persist but are absorbed during development (Slijper, 1962; Peredo et al., 2017), so that they serve as temporary vestiges or atavisms. As these germs fade, they are replaced by arcs of tissue, running along both sides of the rostrum, that generate the alpha-keratin components comprising baleen (Fudge et al., 2009; Szewciw et al., 2010; Thewissen et al., 2017). Basically, the two primary paired components are almost exactly like human hair and fingernails. These elements are arrayed as a sandwich (Figure 1), with two flat outer layers of nail-like sheets (Forslind, 1970) surrounding a core of long, hollow “horn tubules” that dangle down ventrally from the palate (Werth, 2000). The interior of every plate consists of an array of one to two dozen variably-sized tubules within a matrix of intertubular keratin (Werth, 2017). This intertubular keratin matrix binds all components together and acts as an important if much lesser third ingredient for the “sandwich” (Werth et al., 2018b). In this way, baleen’s construction is reminiscent of a fiberglass composite material, with alternating flat sheets and long, narrow fibers (Greenberg and Fudge, 2012). Together, these components provide baleen with unparalleled flexibility and rigidity, combining to create a highly pliant and bendable yet stiff and resilient material (McKittrick et al., 2012) that absorbs intensely high forces and pressures yet bounces back to its original form, all the while resisting crack propagation (Werth, 2013; Werth et al., 2018b).
Nonetheless, baleen’s composite construction not only enables but indeed facilitates its erosion into a tattered curtain of exposed horn tubules that are revealed, like the frayed border of a worn fabric, as hair-like fringes dangling at the exposed, eroded edges of each baleen plate (Werth, 2017). These fringes, also called baleen bristles, not only resemble hairs but in fact are created and grow in virtually the same way as human scalp and body hairs (Fraser et al., 1972, Fraser et al., 1976; Hearle, 2000; Feughelman, 2002), as tubes of alpha-keratin that emerge from a follicle where dermal and epidermal generative cells interact (Thewissen et al., 2017). Because these hair-like fringes run solely dorsoventrally, from a plate’s gingival origin to its ventral-most vertex, transverse (mediolateral) cracking of any baleen plate is prohibited even as longitudinal cracking is facilitated. Not only does this make baleen a strong, resilient material, but even more importantly it creates a more elaborate, less coarse filter that consists not only of the flattened baleen plates (major and minor) but also the much smaller, finer, more flexible fringes (Pfeiffer, 1992; Young, 2012; Jensen et al., 2017; Loch et al., 2020; Vandenberg et al., 2023).
Several routine filtering stresses combine to facilitate baleen’s erosion into fringes (Werth et al., 2016b). These include prey accumulation, seawater flow, and mechanical abrasion from adjacent impinging oral tissues, particularly the tongue and lips (Werth, 2001). Not only do these forces produce the filter’s final structure, but they also contribute to the erosive loss of distal-most fringes, which often appear in whale stomach contents and feces (Werth et al., 2020). Erosive loss is balanced by an equivalent proximal growth of new plate and tubular material (Ruud, 1940), such that the ever-growing baleen filter maintains a near-constant size and shape despite constant turnover from continued wear and tear of feeding (Werth et al., 2021).
Mysticetes are, like many animals, opportunistic and resourceful foragers whose diets are frequently broad (Slijper, 1962; Gaskin, 1982). However, in mysticete taxa whose diet habitually includes very small prey items (such as rice grain-sized copepods), the eroded fringes are measurably longer, finer (i.e., with smaller diameter), and denser (i.e., more eroded fringes per cm of plate) than the coarser fringes of species that typically feed on schooling fish or large zooplankton such as finger-sized krill (Werth, 2000, Werth, 2001). Whale species with fine filters include the balaenid (bowhead and right) whales and the sei whale, Balaenoptera borealis, of the Family Balaenopteridae (Kawamura, 1974, Kawamura, 1980; Brodie and Vikingsson, 2009; Horwood, 2017; Werth et al., 2018a; Segre et al., 2021).
Recent analyses have determined that baleen’s flexibility is tempered by the inclusion of mineral salts, particularly calcium, which act to stiffen the filtering plates (Pautard, 1963; Szewciw et al., 2010). Mineral content varies by species and diet, ensuring that baleen of whales that habitually feed on tiny prey is more flexible, creating an overall less porous filter (Werth et al., 2018a). Other studies on baleen’s physicochemical properties have demonstrated that baleen is generally hydrophilic (enabling penetration of water, which in turn facilitates flexibility and prevents breakage; Werth et al., 2016a) and largely oleophobic (resisting penetration of oil, which is advantageous for species that live near natural submarine petroleum seeps or man-made oil drilling platforms; Werth et al., 2019a). Baleen has, however, been found to be susceptible to ocean acidification caused by carbon emissions (Werth and Whaley, 2019). Baleen’s filtering function is also highly dependent on its remarkably pliant and rubbery zwischensubstanz, the tough, gum-like gingival tissue from which baleen emerges and which surrounds and embeds all plates in the palate. The zwischensubstanz resists shear forces and crack or tear propagation, enabling the filter to withstand powerful flows as it separates food from water (Pinto and Shadwick, 2013; Werth et al., 2019c).
Because baleen grows continually at a generally predictable rate (Werth et al., 2021), and because it, like mammalian hair and nails, therefore encompasses a “snapshot” of a few years of a whale’s life, baleen has proven useful in physiological research (Werth et al., 2020). This is even more valuable considering how well baleen (again, like other keratinous tissues) retains both endogenous and exogenous substances within a whale’s body, most notably hormones, isotopes, and seawater contaminants (Caraveo-Patiño et al., 2007; Pomerleau et al., 2018). Therefore, baleen has become highly advantageous for biologists whose studies focus not on filtration but instead on endocrinological, isotopic, and toxicological or pollutant research.
So what, then, constitutes the actual baleen filter? The plates alone? Fringes? Racks? All of the above? Although this might appear at first glance to be a simple question, it is one of the lingering mysteries of the comprehensive baleen filtration system, and the subject of ongoing research. Clearly, there is much room for judgment, as the composition of the complete baleen filter depends on one’s perspective. When considering an entire baleen rack and its constituent major/minor plates and eroded fringes, baleen acts both structurally and functionally as a complex metafilter comprising multiple components with differing morphological and biomechanical properties (Pivorunas, 1976; McKittrick et al., 2012; Loch et al., 2020). By “metafilter” we mean that each component serves as its own kind of filter, but they work together as a hierchically complex, integrative system. Together, the larger plates and smaller eroded fringes produce a dual-phase (i.e., plate plus fringe) filter, with fringes capturing smaller particles than the larger particles trapped by plates, and with a combined surface area much larger than would be contributed by the flat plates alone (Werth et al., 2018a). This surface area includes the smooth planar surfaces of baleen plates, the tattered region of eroded baleen fringes along each individual plate, and the combined dimensions of each exposed hair-like fringe. Calculations of overall filter area range from roughly 2-4 m2 in most whales to >6 m2 in large balaenids (Table 1), if considering only the surface area of the medial mat of eroded fringe hairs (Kawamura, 1974; Werth et al., 2018a). If the planar surfaces of all plates and dimensions of individual fringes are also included, the total surface area ranges from ~30 m2 in smaller whales to >150 m2 in large balaenids (Werth et al., 2018a).
At this point, it is best to conclude that far from being a simple system, baleen filtration instead acts on micro- to macroscopic scales as a variable metafilter, with each component—fringe, plate, and whole rack—contributing its own function to the entire baleen filtration system (Pivorunas, 1976; Sekiguchi et al., 1992; Vandenberg et al., 2023). Depending on the size of filtered items (1 mm copepods to 20 cm fish) and parameters of filtration (e.g., flow speed), different component elements are involved.
3 Varied filtration modes from diverse mysticete feeding ecology
In addition to the dynamic intricacy arising from the baleen metafilter’s physical structure itself, a second level of complexity arises from differential employment of baleen filters during feeding, which varies widely by taxa (Werth, 2000; Young, 2012). As outlined in Table 1, hinted at in the preceding section, and dealt with in detail in this section, different mysticete families use their filters for different types of bulk prey collection and separation from seawater (Figure 2). In essence, the three main living families employ wholly different foraging strategies (Table 1).
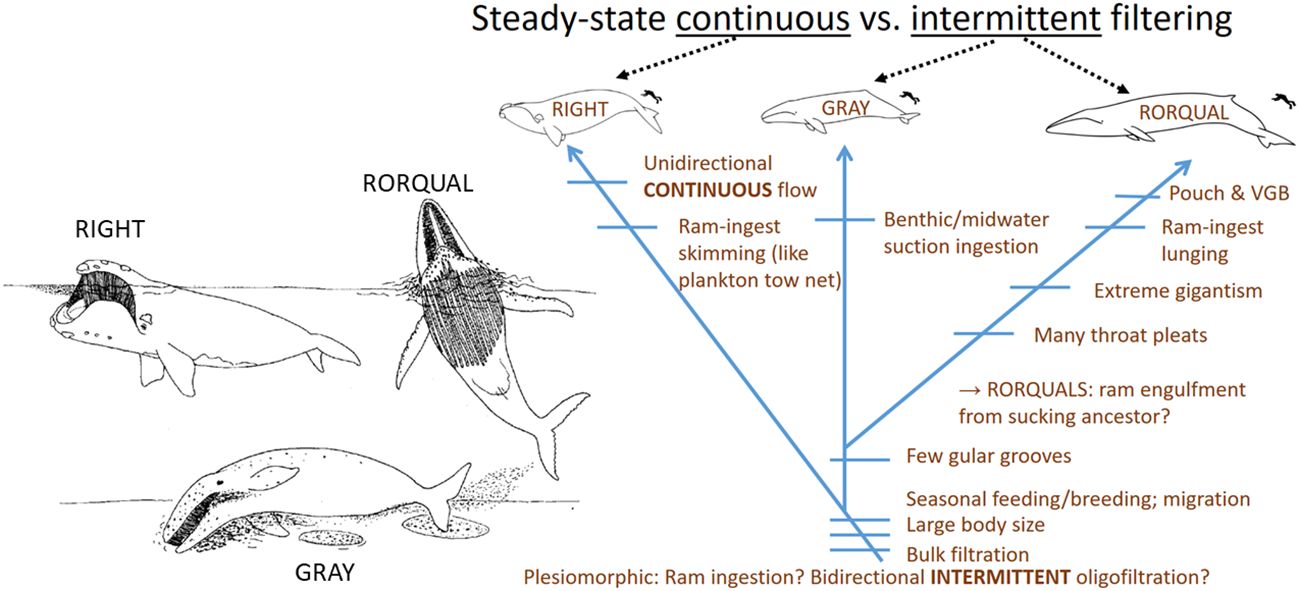
Figure 2 Baleen is used for steady-state continuous filtration in bowhead and right whales, or intermittent filtration (processing a single discrete mouthful of prey-laden water) in gray whales and groove-throated rorquals. Cross-sections through mouth at top left indicate that oral morphology parallels these feeding types. There is much speculation but no consensus as to which type of foraging filtration arose in the earliest baleen whales and is ancestral to other types. There is also debate about when gigantism arose in mysticetes; some sources (e.g., Bisconti et al., 2021, Bisconti et al., 2023) argue that at least in right whales (Balaenidae), smaller body size is plesiomorphic.
The most fundamental division of mysticete feeders involves continuous versus intermittent filtration (Tomilin, 1954; Nemoto, 1959; Slijper, 1962; Nemoto, 1970; Gaskin, 1982; Sanderson and Wassersug, 1990, Sanderson and Wassersug, 1993; Werth, 2000). Continuous filtration operates over extended bouts of time (typically several minutes of deployment) as a steady-state process (Werth, 2004), the way plankton tow nets are used, except that whales push rather than pull the filter via forward locomotion from steady fluking. In contrast, intermittent feeders engulf and process a single mouthful of prey-laden water at a time (Goldbogen et al., 2017b).
Balaenid (bowhead and right) whales are typically ram skimmers that use continuous bulk filtration to filter small planktonic prey wherever prey accumulate: at the surface or all levels of the water column, including near the bottom (Mayo and Marx, 1990). As the mouth opens to expose the baleen filter, an incurrent flow of prey-laden water enters the mouth between paired racks (Figure 2). Only balaenids have a true subrostral gap between left and right racks (Werth, 2004). Balaenids also have high, semicircular lips extending above the lower jaw, with a port-like opening just behind the lips and under the eyes for steady excurrent flow (Werth and Potvin, 2016). The resulting scoop-like head can comprise a third of the length of a balaenid’s plump body. After flowing through the center of the mouth, water flows laterally through each rack, then through a gutter-like fold, the orolabial sulcus, between the lip and lateral rack surface (Lambertsen et al., 1989; Potvin and Werth, 2017). In addition to laterally supporting and constraining the baleen racks, the lips may be especially important in generating and modifying flow regimes (Werth et al., 2018a; Werth et al., 2019b); their positioning should be the focus of further flow experiments and field observations. The balaenid tongue may preferentially direct flow toward either rack (Werth, 2007; Werth and Crompton, 2023); it also, without moving, sets up a flow regime that channels flow toward each rack (Potvin and Werth, 2017; Werth and Sformo, 2020). Balaenids typically swim at about 0.7-1.0 m/s when filtering prey (van der Hoop et al., 2019; Werth and Sformo, 2020). They close the mouth, apparently to swallow a slurry of accumulated prey, at regular intervals. Data from tags temporarily affixed to whales reveal that balaenids usually feed during dives of approximately 15 min (Werth and Potvin, 2016; Werth and Sformo, 2020). In bowheads, the mouth closes for about 10 s every 2-3 min (Simon et al., 2009), whereas in right whales feeding on concentrated prey, the mouth closes for ~3 s every 50 s (van der Hoop et al., 2019); these behaviors vary in duration with prey density. Fluking data from tags indicate that gape opening to expose baleen for filtration engenders a notable rise in drag (Nowacek et al., 2001; Potvin and Werth, 2017). Drag has been calculated to increase to five times the baseline level in foraging North Atlantic right whales (Nousek-McGregor, 2010; van der Hoop et al., 2019) and sixfold in bowhead whales (Simon et al., 2009). These forces are estimated at 10-100 kN, or 0.1-1 kN per metric ton of body mass (Potvin et al., 2020). As a consequence of this increased drag, swim speed during filtration immediately drops (even with notably increased fluking rates) by 25% in right whales and 40% in bowheads (van der Hoop et al., 2019; Werth and Sformo, 2020). Little is known of feeding in the smallest mysticete species, poorly understood pygmy right whales, Caperea marginata, but it is presumed, based on aspects of the baleen filter and related oral morphology, that this species also collects and filters tiny zooplankton via skimming (Kemper, 2017; Werth et al., 2018a).
Foraging in the rorqual or “groove throated” whales, so named for their prominent pattern of ventral throat pleats (Shadwick et al., 2013), occurs in an entirely different manner generally described as “lunge feeding” (Figure 2). These diverse whales of the Family Balaenopteridae, which includes the largest baleen whales (blue and fin; Balaenoptera musculus and physalus) and much smaller minke whales (B. acutorostrata and bonaerensis), as well as the familiar humpback whale (Megaptera novaeangliae), use intermittent instead of continuous filtration. A single mouthful of prey are engulfed, usually in an energetic, acrobatic lunge or gulp at the surface or at depth. Water is then expelled by contraction of muscles underlying the ventral groove blubber or VGB (Orton and Brodie, 1987; Goldbogen et al., 2010; Shadwick et al., 2013; Werth and Ito, 2017; Kahane-Rapport and Goldbogen, 2018; Pyenson, 2018) and prey are swallowed. Although rorquals normally display sleek bodies adapted for rapid locomotion (both for long migrations and predatory lunges), when they engulf a capacious mouthful of prey-laden water, they briefly assume a bloated “tadpole” shape, as the accordion-like throat pleats expand over the entire ventral region, spreading posteriorly to the umbilicus (Werth et al., 2019b). Adult rorquals have a floppy, flaccid tongue (Werth and Crompton, 2023), which invaginates into a hollow interior space, the cavum ventrale, to accommodate the massive temporary influx of water, which may involve over 100 m3 (100,000 L; Werth, 2013).
Apart from stealthy “trap feeding,” in which a stationary whale at the surface holds its jaws open and waits as prey accumulate inside the mouth (McMillan et al., 2019), rorquals generally lunge via rapid forward locomotion. Thus both the entry of water/prey and subsequent expulsion of water are rapid, forceful events (Simon et al., 2012), unlike in balaenid skim feeding (Simon et al., 2009; Werth and Potvin, 2016). However, both balaenid skim and rorqual lunge feeding depend on locomotion-based ram ingestion (apart from rare cases of passive trap feeding). Sei whales, B. borealis, display a morphotype somewhat intermediate between balaenids and other balaenopterids (Werth et al., 2018a), and are known to switch facultatively between lunge feeding and balaenid-style skim feeding depending on targeted prey (Segre et al., 2021).
Scalable parameters of rorqual lunge feeding vary somewhat by species and size yet display remarkable ecological/behavioral and morphological/physiological consistency in traits ranging from gape angle and duration to timing of water filtration and expulsion (Goldbogen et al., 2006, Goldbogen et al., 2007, Goldbogen et al., 2011; Goldbogen et al., 2012a, Goldbogen et al., 2012b; Potvin et al., 2009, Potvin et al., 2012; Goldbogen et al., 2015; Cade et al., 2016; Kahane-Rapport et al., 2020; Potvin et al., 2020, Potvin et al., 2021). Generally, all engulfed water is expelled from the expanded oral and throat pouch within about 20 s (Goldbogen et al., 2017b). Unlike balaenids, balaenopterids have only a small lower lip rising above the mandibles, but this may help to hold the baleen filter and keep it from bulging outward as strong forces and pressures accrue during the brief yet powerful burst of water expulsion (Werth, 2013). Again, the extent to which the lips play a direct or indirect role in filtration remains unknown, and should be the focus of future study.
A third kind of mysticete foraging, and second kind of intermittent baleen feeding (Table 1), occurs in gray whales, Eschrichtius robustus. These typically employ intraorally-generated suction, created by rapid tongue depression and retraction, to ingest benthic invertebrates, although gray whales, like other mysticetes, display ecological and behavioral versatility to exploit multiple food resources (Kasuya and Rice, 1970; Rice and Wolman, 1971; Ray and Schevill, 1974; Nerini, 1984; Sumich, 2001; Woodward and Winn, 2006; Young et al., 2015; Webber et al., 2024).
Although this general three-way pattern of mysticete feeding is widely understood (Pivorunas, 1979; Berta et al., 2015, Berta et al., 2016), much speculation surrounds the origins of baleen from early toothed ancestors and the presumed original type of mysticete feeding (Fitzgerald, 2010; Boessenecker and Fordyce, 2015; Tsai and Fordyce, 2015; Marx et al., 2016a; Geisler et al., 2017; Gol’din and Startsev, 2017; Lambert et al., 2017; Peredo et al., 2017; Hocking et al., 2017b; Fordyce and Marx, 2018; Ekdale and Deméré, 2021; Gatesy et al., 2022). Notably, whether the earliest baleen use involved intermittent or continuous filtration remains unknown, despite much conjecture. Several studies have concluded that early baleen use involved suction rather than ram ingestion. The phylogenetic placement of gray whales close to (indeed, within) balaenopterids is now well established (Gatesy et al., 2013), but how their feeding style arose is uncertain. It is possible that the first filtering mysticetes fed similarly to modern gray whales; alternatively, gray whale suction feeding may have arisen as a specialization from lunging ancestry. It is also possible that the earliest baleen filtration involved something akin to the continuous skimming of modern balaenids. A growing record of diverse yet somewhat contradictory fossils raises more questions than answers (Mchedlidze, 1984; Thewissen, 1998; Kimura and Ozawa, 2002; Bisconti and Varola, 2006; Thewissen et al., 2009; El Adli et al., 2014; Berta et al., 2015; Collareta et al., 2015; Berta et al., 2016; Marx et al., 2016b; Werth and Marshall, 2023).
For the purposes of this review paper, the most important point to be made here is that baleen appears to have been a spectacularly successful key innovation, leading, roughly 28 million years ago (Bisconti et al., 2023), to an adaptive radiation of diverse stem and crown Mysticeti (Thewissen, 1998; Marx et al., 2016b), with each lineage evolving its own baleen filter arrangement with plates and fringes of varying number, size, shape, and material properties (Nemoto, 1970; Werth et al., 2018a; Marshall and Pyenson, 2019). Extinct and extant baleen whales have used, and continue to use, their keratinous oral filters in remarkably varied ways. Consequently, baleen filtration systems of different mysticete taxa are subjected to radically differing design requirements ranging from brief bursts of rapid, high volume flow to sustained periods of low-speed flow exposure engendering very high drag forces (Fitzgerald, 2010; Marx et al., 2016a, Marx et al., 2017; Potvin et al., 2020). The bottom-line conclusion is that there is no unified pattern of baleen filtration because there is no single strategy of mysticete filtration. Instead, there are three main strategies (Figure 2; Table 1), with further elaborations for taxa such as sei and pygmy right whales (Sekiguchi et al., 1992; Brodie and Vikingsson, 2009; Werth et al., 2018a). Baleen has evolved to fit a wide variety of dynamically variable situations both within and between different species and lineages.
4 Flow-dependent porosity determined by varying parameters
In addition to baleen’s structural and functional diversity arising from the structural complexity of the filter and ecological array of its usage, a third layer of variability (Figure 3) has been made apparent by controlled laboratory experiments (e.g., Werth, 2013; Werth and Potvin, 2016; Potvin and Werth, 2017, Potvin and Werth, 2024) confirming that parameters of baleen filtration vary according to operating conditions. Most notably, these involve flow direction (angle of attack), speed, and volume. Further, because of the baleen’s structural complexity (Werth, 2017), these parameters vary by location within the filter (Figure 4), namely: at different sites 1) within a rack (anteroposteriorly and mediolaterally), 2) along an individual plate (dorsoventrally, from palatal origin to the triangular vertex corresponding to the oldest exposed part of a plate), and 3) along a fringe, from where a fringe connects to a plate along its distance to the free tip. The upshot of these variables is that baleen’s porosity (Matyka et al., 2008; Vogel, 2013; Potvin and Werth, 2024) is flow-dependent and also region-dependent (Figure 4; Werth, 2013; Potvin and Werth, 2024). Unlike a simple kitchen colander or other strainer, baleen does not demonstrate fixed porosity (Werth, 2019).
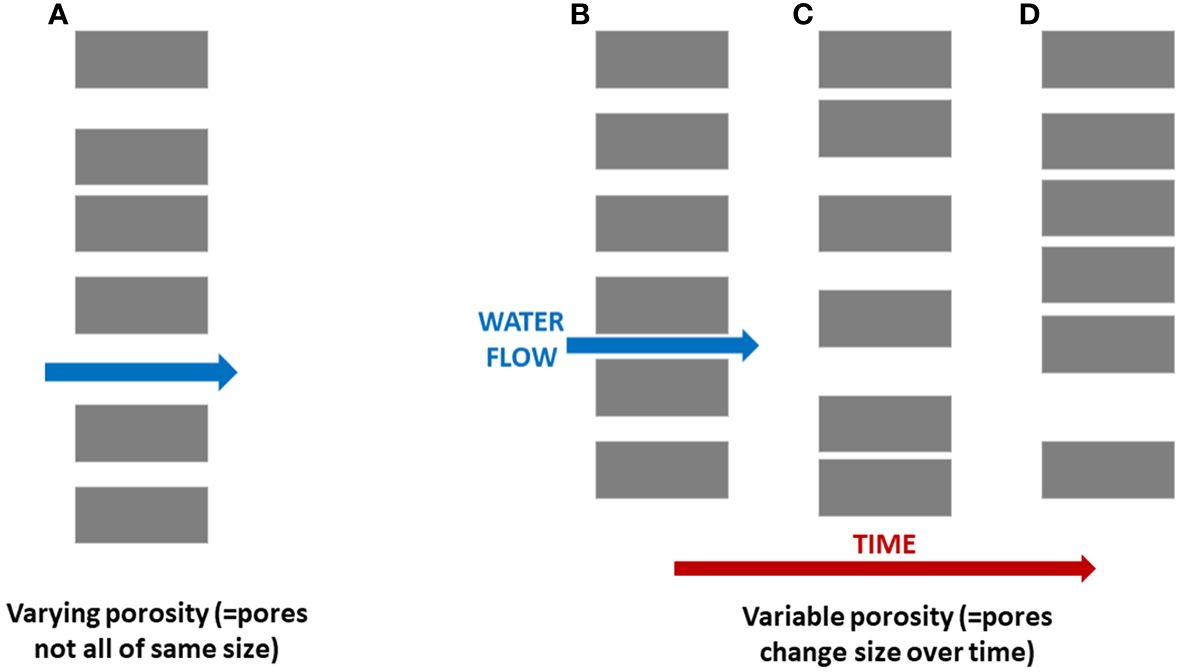
Figure 3 Research has revealed that unlike a simple sieve, baleen operates as a highly dynamic filter whose porosity is both spatially varied (A) and temporally variable (B–D) as well as varied by species. Gray rectangles indicate sections through plates or fringes of baleen, with white gaps representing porous spaces in between.
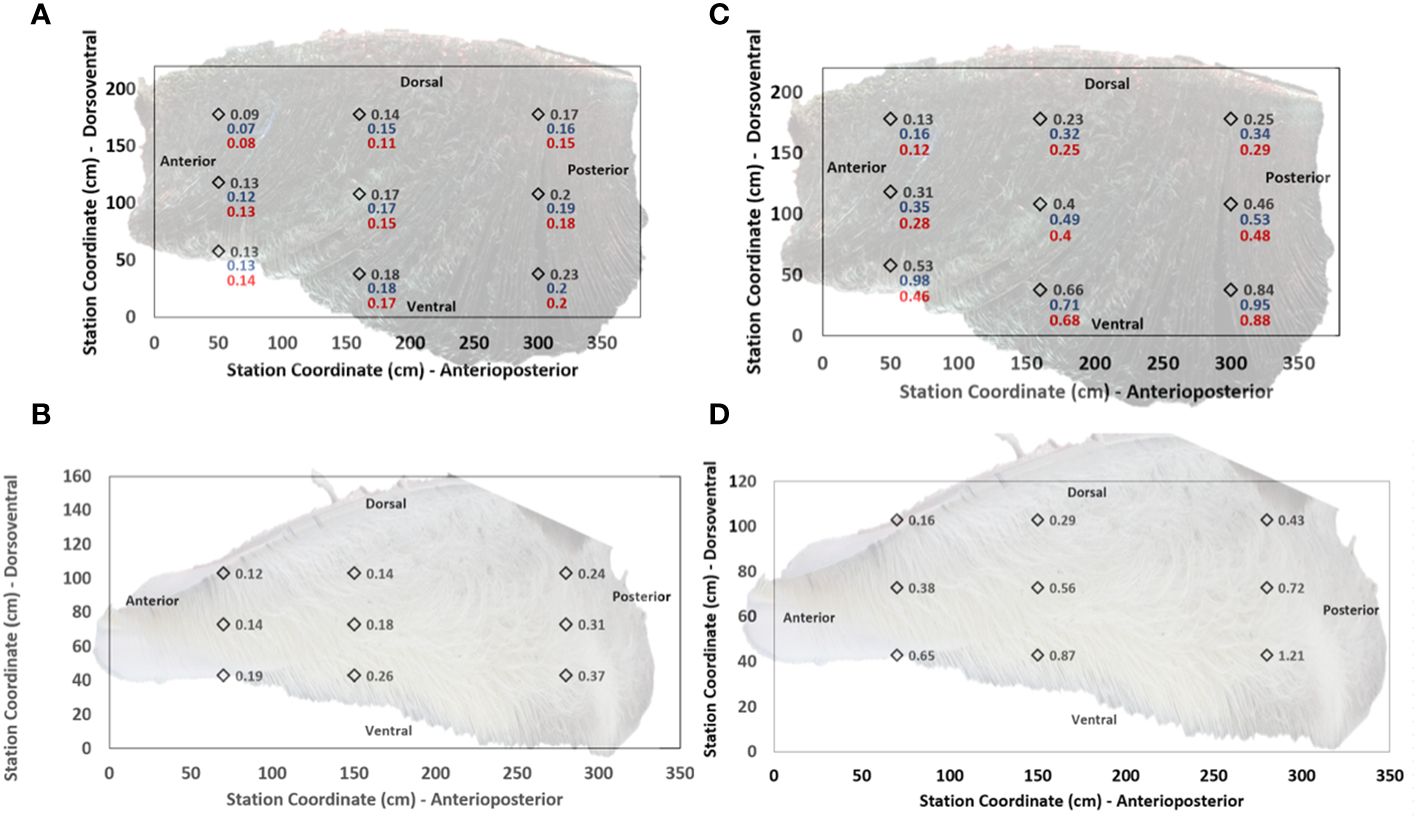
Figure 4 Porosity varies by locations on the interior (medial) fringed mat of baleen racks, as indicated on samples collected from bowhead (A) and fin (B) whale specimens in a flume (Potvin and Werth, 2024). Diamonds indicate approximate locations on a rack, with background photographs rescaled to guide the eye. Labels correspond to porosities measured at flume speeds of 0.75m/s (black print), 0.50m/s (blue) and 0.25m/s (red). Typical uncertainties fall within the SD of ± 0.04 (bowhead) and ± 0.09 (fin) calculated in the observables’ average over all anatomical stations. Right panels show through-mat flow speeds (cm/s) versus position on bowhead (C) and fin (D) whale racks (with symbol conventions as in panels (A, B)), calculated at a benchmark pressure gradient set equal to the product of water density, acceleration of gravity and local mat thickness (Potvin and Werth, 2024).
This conclusion is at once both obvious (upon reflection) but at the same time unexpected given traditional views of baleen’s function as a sieve (Sanderson and Wassersug, 1990, Sanderson and Wassersug, 1993). Further, until recently the structural intricacy of the baleen filter has been greatly underestimated (Young et al., 2015; Jensen et al., 2017; Vandenberg et al., 2023), as has the array of foraging conditions in which baleen filters are used (Goldbogen et al., 2017b; Goldbogen and Madsen, 2018; Goldbogen et al., 2023). In addition to strong speculative inference from such morphometric and ecological analyses, two additional sources of robust data have revealed much detail about baleen filtration. The first involves field data from biologging tags (archival and telemetric) temporarily affixed via suction cups to the bodies of living whales. These continue to yield great insight into how whales filter prey during feeding (Goldbogen et al., 2017a). Tag data have shown for example that whales control body position (roll, pitch, yaw), open and close the mouth for specific durations, swim at certain speeds, and feed at various depths during filtration, all of which have implications for how the baleen filter operates (Goldbogen et al., 2013; Goldbogen et al., 2017b, Goldbogen et al., 2023).
The second source of data involves less striking but equally valuable laboratory experiments using baleen tissue in circulating flow tanks (also called flumes; Mayo et al., 2001; Werth and Potvin, 2016; Potvin and Werth, 2024). Because of the large size of actual baleen plates, researchers generally cut small sections from plates and assemble them to create “mini-racks,” although huge flow tanks created for naval architecture studies (i.e., to test fluid dynamics of vessel hulls) have also been used (Werth et al., 2018b). The baleen can then be observed as it responds to moving water, either as water circulates past stationary baleen (Werth, 2013) or as baleen is towed or otherwise propelled through water (Werth et al., 2018b). In this way, changes in baleen form and “posture,” such as bending and spacing between plates and fringes, can be observed, recorded, and analyzed. Additionally, dyes or other materials can be added to water to better visualize hydrodynamics of baleen-water interactions. Small particles such as plastic beads, or even genuine prey items (e.g., copepods or other zooplankton; Fields and Yen, 1997; Werth, 2012) can be introduced to flow to record actual filtration events via cameras placed directly underwater in the flowstream or by outside viewing ports, ideally with ruled grids or other scales to indicate and quantify flow regimes (Werth, 2013). One way to measure such interactions is by analyzing rates at which baleen plates and fringes capture plastic or prey particles in the water stream; another is to determine the extent to which baleen porosity changes as plates and fringes move within the flow (Figure 5). [Note that “capture” in this sense means that particles are caught by the baleen filter (Shimeta and Jumars, 1991; Potvin and Werth, 2024). Capture could also include ingested particles flow directly into the oropharynx with little or no contact with the filter.] The distance between the free tips of eroded fringes or of another location along fringes (e.g., 1 cm or 10 cm from where it attaches to a plate), defined as interfringe distance (IFD), has provided valuable data about porosity and other filter parameters as variables such as flow speed are experimentally manipulated (Werth, 2013, Werth, 2019).
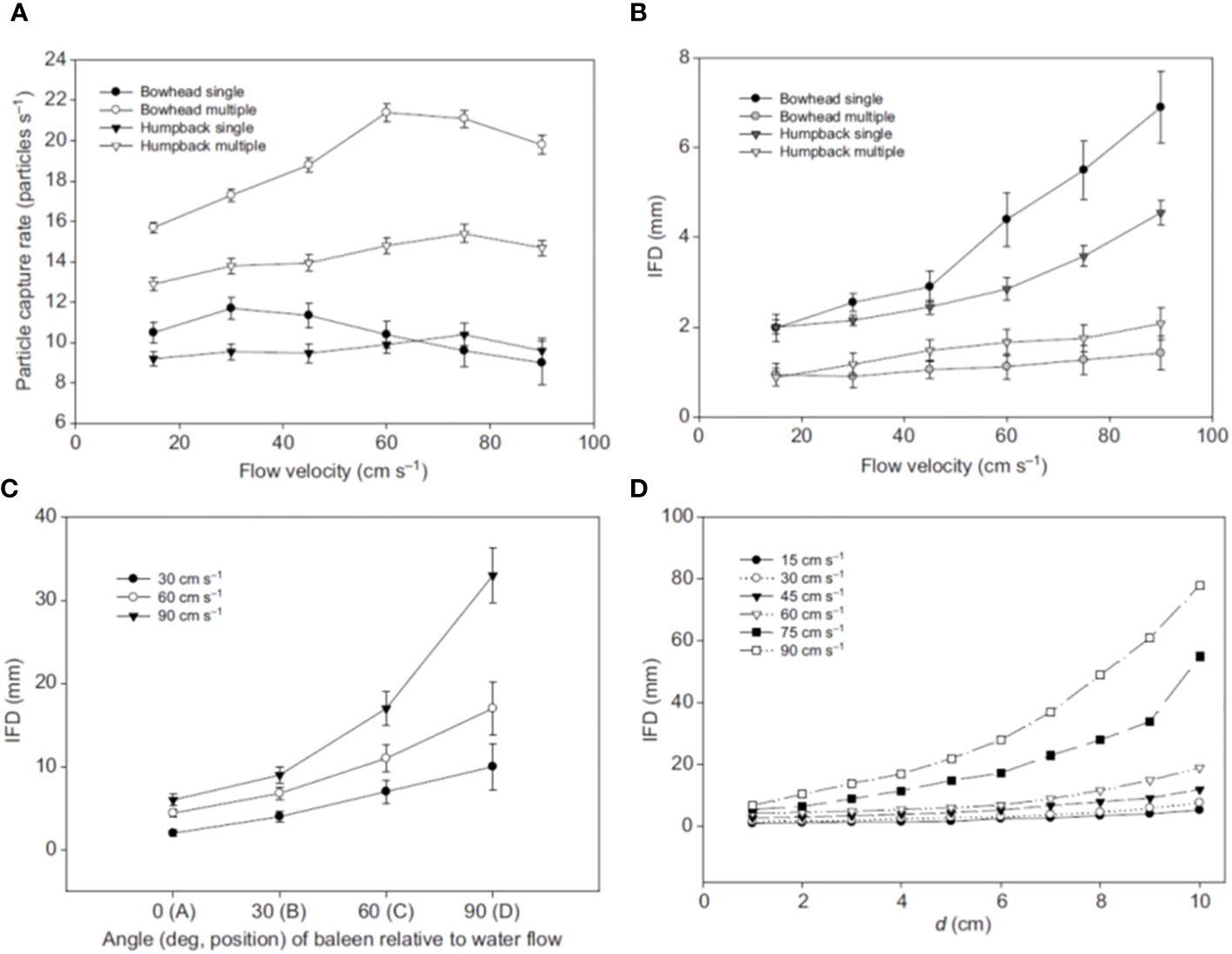
Figure 5 Laboratory flume experiments (from Werth, 2013) with baleen samples show that many factors, including flow parameters (speed, direction, volume flow rate) and baleen’s orientation (angle of attack) and the distance (d) of fringes from plates, all result in different functional outcomes as measured by such parameters as particle capture or inter-fringe distance (IFD). Panel (A) shows particle capture versus flow velocity; (B) shows IFD versus flow velocity; (C) shows IFD versus angle of attack; (D) shows IFD versus distance of fringe from plate.
Such experiments have yielded useful information regarding baleen filtration (Figure 5). They have revealed for example that the direction of water flow, determined by the angle of attack at which plates are oriented relative to incurrent flow, considerably alters baleen’s filtration ability (as revealed by particle and pigment movement and capture). As with other basic aspects of baleen filtration, this is both surprising and unsurprising. On the one hand, it is entirely obvious that flow direction has a marked effect on filtration (Werth, 2013). On the other hand, this is somewhat unexpected given that people historically viewed baleen filtration as a relatively simple, invariant process (Jorgensen, 1966; Croll et al., 2008). Instead, recent research has shown (as outlined in the next two sections of this paper) that flow direction varies throughout baleen filtration by whale species, as well as by location within the rack and throughout the overall time course of an individual bout of filtration.
In addition to variation in filtration according to the flow angle of attack (Figure 5C), flow speed also leads to differences in baleen’s filtering abilities as indicated by mat porosity (Figure 4), particle capture rate (Figure 5A), and IFD, which is a major determinant of porosity (Figure 5B; Werth, 2013; Potvin and Werth, 2024). As flow speed and volume flow rate increase, fringes are generally pushed farther apart, leading to higher IFD (Figure 5B). As noted above, flow speed and volume vary by location and timing within a single filtration event, especially in rorqual lunge feeding but also in balaenid skimming and gray whale benthic suction feeding (Werth, 2019). Experiments have revealed the crucial importance of how free baleen fringes interact between adjacent plates (Werth, 2013; Werth et al., 2018b). If a single plate is tested in a flume, it appears that higher flows, particularly above 60 cm/s, lead to diminished particle capture and higher IFD, meaning the filter is more porous (Werth, 2013). However, when multiple plates are tested together in a “mini-rack” formation (Figure 5), it can be seen that baleen fringes swirling in the flow actually overlap and intermingle to form a denser, less porous mat (Potvin and Werth, 2017, Potvin and Werth, 2024). Apart from the overall lesson that basic experimental design is a key consideration for experimental simulations, the more pertinent conclusion is that IFD is a variable parameter that depends on interaction between multiple plates. Each free, eroded horn tube fringe erupts from a single keratinous plate, but fringes from multiple plates work together to create the structurally and functionally important fringe mat (Werth, 2013; Potvin and Werth, 2017; Werth et al., 2018a; Potvin and Werth, 2024). As described earlier, the total baleen filter is a metafilter that is greater than the sum of its individual constituent components. Once again, mysticete filtration is not nearly as simple as traditionally presumed. Specifically, porosity and other measures of filtration depend not only on baleen’s physical arrangement and dimensions (e.g., length and density of fringes and plates), but also on flow parameters, notably the speed and direction of water flow.
5 Tangential filtration instead of simple throughput sieving (in balaenids and possibly other mysticetes)
Further analysis and ongoing experimentation have revealed that one of the most fundamental ways in which baleen filtration is more complex than commonly regarded involves the basic type of filtration. Traditional accounts depict baleen as a sieve (Slijper, 1962; Werth, 2001). This is true not only of children’s literature, but also of specialized academic works, nearly all of which describe baleen filtration as merely separating prey by size with a passive strainer (Rubenstein and Koehl, 1977). Such passive sieving is presumed to work by throughput (AKA dead-end) filtration, in which prey-laden water flows directly into the filter at a more or less perpendicular angle, thereby collecting and retaining any items larger than the gaps (pores) of the filter, which in this case consist of gaps between baleen plates and/or between fringes (Pivorunas, 1976; LaBarbera, 1984).
Although such a throughput sieve arrangement could work effectively, for several reasons it would not work efficiently for mysticete oral filtration (Werth, 2019). To understand why, it is helpful to consider why throughput filtering is rarely used in commercial filtration scenarios, such as processing to remove particulate matter from beer, wine, juice, or other beverages (Starbard, 2009; Tamime, 2013; Jain and De, 2019). Although such industrial processing seeks to eliminate the solid retentate trapped by the filter (a process called clarification; Sutherland, 2005) and retain/recover just the fluid flowing through it—the opposite of the whale strategy to retain and ingest the filtered solids and expel the watery medium—the overall principles are the same (Blatt et al., 1970). If throughput, perpendicular filtration were used in beverage processing, the filter could readily clog, impeding flow and slowing fluid throughput (and filtration output). Further, the filter might more readily break or otherwise fail and require repairs or replacement, not to mention the fact that repeatedly clearing solid retentate from the filter would be a costly and inefficient chore. Completely replacing the filter, as with coffee machines, is not feasible for whales and seldom a viable option for organismal filters, especially in vertebrates (although filter replacement occurs in larvaceans, caddisfly larvae, some worms and snails, and suspension feeders that continuously produce an internal mucus net to trap prey, including enteropneusts, ascidians, lancelets, and some tadpoles; Hamann and Blanke, 2022).
Tangential filtration, in which the flow strikes the filter at a low angle, reduces or even precludes these problems of solid accumulation, filter clogging, and filter cleaning (Baker et al., 1985; Murkes and Carlsson, 1988; Lu and Ju, 1989; Lu et al., 1993; Belfort et al., 1994; Song and Elimelech, 1995; Vogel, 1996; Zeman and Zydney, 1996; Vogel and Todaro, 1997; Bott et al., 2000; Brainerd, 2001; Sibanda et al., 2001; Ripperger and Altmann, 2002; Espinosa-Gayosso et al., 2012; Makabe et al., 2021). In perhaps its most ideal tangential form, flow runs longitudinally along the filter rather than directly into and through it. In this scenario, called cross-flow filtration, the solid retentate is not allowed to accumulate along the filter; rather, solids are propelled past the filter or, depending on the flow regime (Mei, 1992; Humphries, 2009; Peng and Dabiri, 2009), at the most distal part of the filter, where they can be more easily dealt with. In cross-flow filtration, the filter rarely if ever clogs (and if clogging occurs it is delayed substantially; Ripperger and Altmann, 2002; Makabe et al., 2021), and the filter is subjected to less potential damage from high pressures or other physical forces, meaning the filter likely lasts longer (Brainerd, 2001). Thus apart from less filter wear or damage, separation of solid and fluid is more efficient with cross-flow than throughput filtration in terms of delayed or absent clogging, and thus more fluid processed per unit time (Potvin and Werth, 2017). For whale feeding, all these benefits would apply (Potvin and Werth, 2017), with the further benefit that the solid retentate—that is, the collected prey items, which recall are the desired outcome of whale filtration, unlike filtration of commercial beverages or other products—accumulate at or near the excurrent flow output (Werth and Potvin). In the case of the whale mouth, this is right at the entrance of the oropharyngeal opening, where prey are swallowed. Accumulation of a bulk slurry of small prey items that have been efficiently separated from undesirable seawater is an optimal form of filtration (Zhu et al., 2020a, Zhu et al., 2020b, Zhu et al., 2021, Zhu et al., 2023). Cross-flow filtration feeding has been well documented in bony fish (Langeland and Nost, 1995; Goodrich et al., 2000; Brainerd, 2001; Cheer et al., 2001; Sanderson et al., 2001; Smith and Sanderson, 2008; Paig-Tran et al., 2011; Cheer et al., 2012; Sanderson et al., 2016; Witkop et al., 2023), sharks (Sims, 2008; Motta et al., 2010; Wegner, 2015), and rays (Paig-Tran et al., 2013; Paig-Tran and Summers, 2014; Divi et al., 2018).
Such cross-flow filtration would be ideal for continuously feeding balaenid whales, which take in a steady incurrent stream of pre-laden water for a minute or several minutes (depending on prey type and density) before closing the mouth and swallowing accumulated prey; filtration in other (non-balaenid) whales will be covered later in this section. The anatomy of bowhead and right whales is well suited to longitudinal cross flow, with the subrostral gap between baleen racks serving as an input orifice, and paired, “jetport”-like excurrent openings posterior to the large semicircular lips and just below the eyes (Werth, 2004). In this pipe-like flow system, water enters, then divides into a Y-shape to flow along and eventually through either the left or right baleen rack (Figure 6). Water flows through the anterior region of the filtering baleen rack, and then flows along a constrained channel, the gutter-like orolabial sulcus, lateral to the rack but medial to the lip (Figure 6A). As water exits the oral cavity over the entire filter surface, longitudinal flow slows posteriorly (i.e., as it approaches the oropharynx). This makes the accumulated bolus easier to swallow; not only is it devoid of water but it is also moving slowly (Werth and Potvin, 2016).
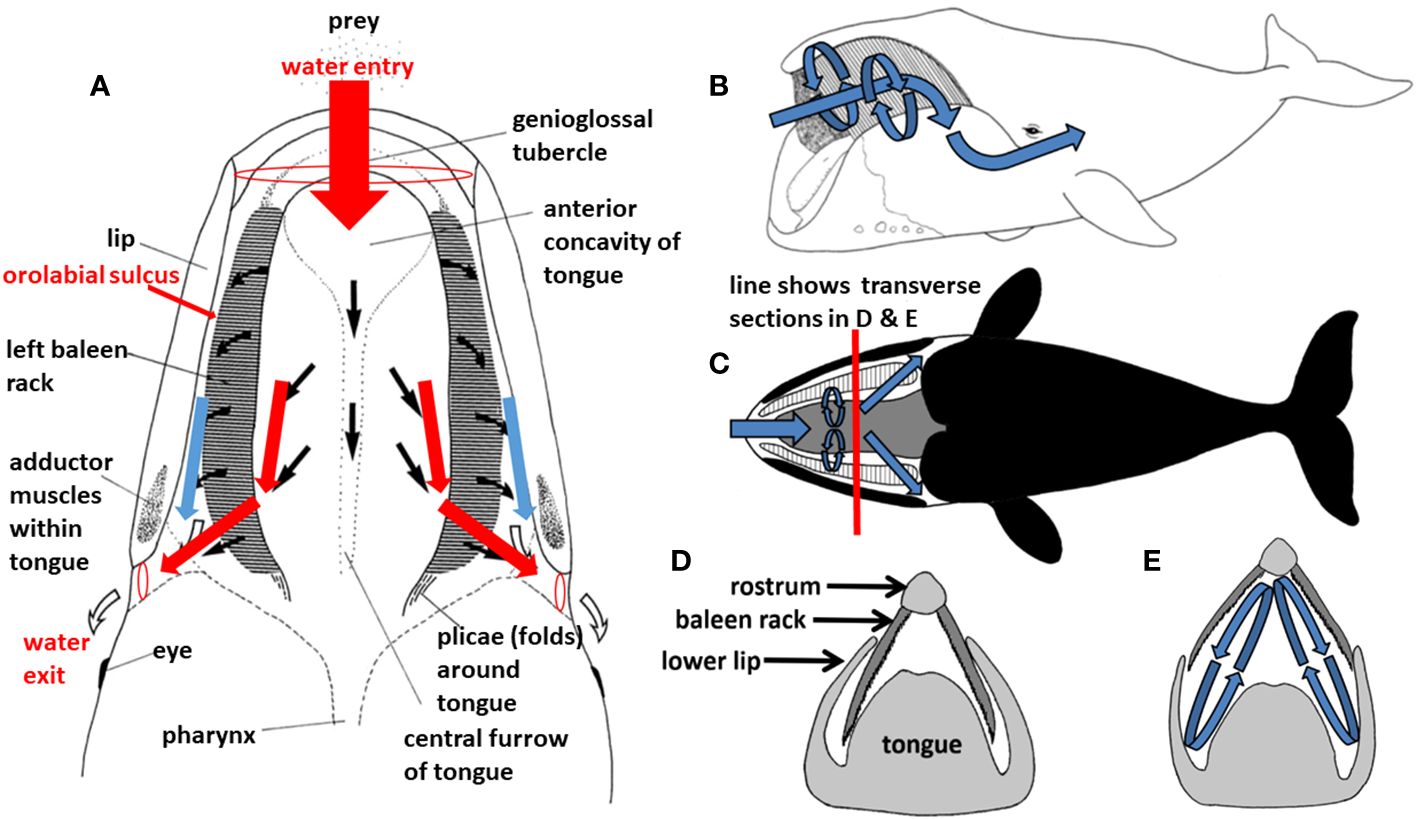
Figure 6 During filter feeding in a balaenid whale ((A), showing dorsal view of mouth in cutaway frontal section), prey-laden water flows continuously in branched Y-shaped pipe-like fashion, generating Bernoulli and Venturi effects. This in turn presumably creates helical vortices (B–E), such that water runs tangentially in cross-flow along rather than perpendicularly through baleen.
The very large (up to 1m high and wide and 4+m long), firm muscular tongue of all balaenid species also channels flow, dividing it and directing it into either baleen rack (Werth, 2000; Werth and Crompton, 2023). Lambertsen et al. (1989) speculated that this intraoral morphology might create a Bernoulli effect, as flow speed increases due to narrowing of the pipe-like path, and that this might further generate a Venturi effect to pull water from the center of the mouth, along the tongue, through baleen. These hydrodynamic effects could also slightly diminish pressures where water enters at the anterior of the mouth, perhaps not sufficiently to generate truly notable subambient suction pressures, but at least enough to obviate an anterior compressive bow wave that might physically disperse small prey and/or warn them of an approaching whale. Flow experiments (Werth, 2004, Werth, 2013; Werth and Sformo, 2020) using pressure transducers and videorecording of particles and prey (Werth, 2012) have confirmed that these limited yet real pressure effects in laboratory settings (Figure 7), and tantalizing photographs and video recordings of whales foraging at sea (Werth and Potvin, 2016; Werth and Sformo, 2020; Werth and Crompton, 2023) have likewise supported conclusions of Bernoulli and Venturi effects.
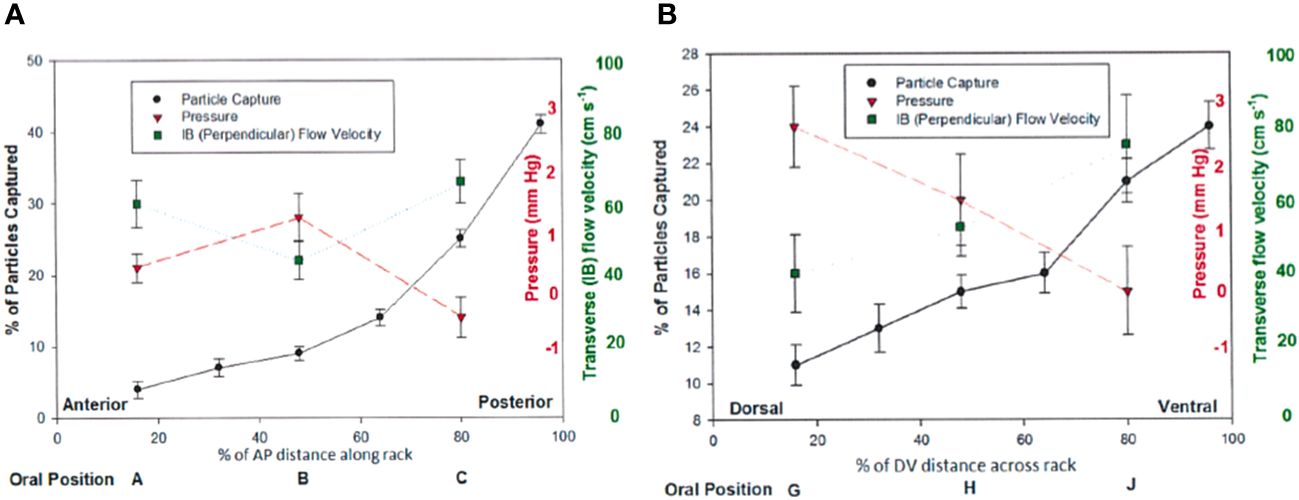
Figure 7 Results from flume experiments (Werth and Potvin, 2016) show differential particle capture, pressure, and perpendicular flow speed effects along both the anteroposterior (A) and dorsoventral (B) dimensions of a full baleen rack, with greater transverse flow and particle accumulation at the posterior end of a full baleen rack, supporting the hypothesis of cross-flow rather than throughput filtration.
In addition to the likely longitudinal (anteroposterior) aspect of balaenid cross-flow filtration, there are additional presumed dorsoventral elements of a balaenid cross-flow regime that would involve generation of helical vortices within the mouth (Figure 6). Such vortices, again created by steady-state flow through balaenid oral geometry, would remain stable for the duration of continuous skimming. There is strong experimental laboratory flume support for dorsoventral cross-flow and vortical flow generation (Werth, 2004, Werth, 2013; Werth and Potvin, 2016). Set up by anteroposterior (AP) flow along the tongue (APT) and lip (APL), this would create sustained helical cross-flow running along each baleen rack from front to back and top to bottom (Figure 8). Although conjectural, we speculate that the minor baleen plates might assist in generating such helical flow. They are well suited to tripping flow and creating a zone of low pressure along the palate, close to the rack’s medial edge. This effect could impart an upward component to the flow, facilitating vortex creation.
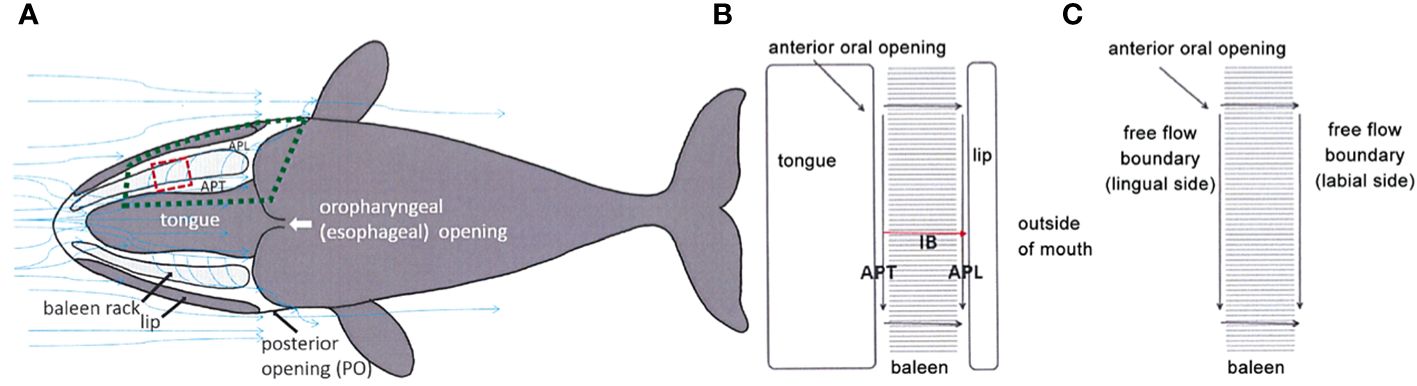
Figure 8 Experiments (Werth and Potvin, 2016; Potvin and Werth, 2017) simulating continuous flow through the balaenid mouth (A) confirm that solid structures on either side of the baleen rack (B) create paired anteroposterior (AP) flow channels along the medial tongue (APT) and lateral semicircular lip (APL) to promote cross-filter flow (C).
Again, this flow regime would most effectively separate prey from water without clogging but with prey accumulation directly on the root of the tongue, where it could be readily swallowed by lingual retraction (Potvin and Werth, 2017). Indirect evidence for this arrangement comes from necropsies on stranded whales or those that die rapidly from ship strikes or other trauma. Despite balaenid feeding on tiny crustacean arthropods (mainly copepods, mysids, amphipods, and euphausiids) with numerous thin, branched exoskeletal appendages that might readily snag in the fine, hair-like fringes of the balaenid filter mat, dead whales are never found with prey items lodged within the baleen filter (Werth and Potvin, 2016).
Although this cross-flow filtration concept has been best considered in balaenid (bowhead and right) whales, it could conceivably apply to all mysticetes (Potvin and Werth, 2017), including intermittently feeding rorqual and gray whales, although likely to a lesser extent. We must note that most recent accounts of baleen filtration (e.g., Hamann and Blanke, 2022; Vandenberg et al., 2023) rely on the notion of throughput filtration in rorquals. However, ongoing flow experiments suggest that at least some flow during rorqual feeding, particularly during latter stages of filtration and water expulsion, involves varying flow pathways that might indicate some cross-flow rather than throughput filtration, and we expect this to be addressed in future publications. Intraoral helical vortices like those envisioned within the balaenid mouth (Figure 6) could likewise form within the mouth of all whales during filtration. This is especially true considering the growing realization that multiple distinct phases of flow are apparent within a single mysticete feeding event, as outlined in the following section.
6 Discrete filtration phases within a single rorqual feeding event
As explained in the first section of this review, traditional accounts of mysticete feeding are greatly simplified and mainly focus on the tongue’s role in expelling water from the oral cavity. However, application of modern technologies, notably photography from aerial drones and underwater cameras (Werth et al., 2019b), including cameras and other instruments on digital biologging tags), have revealed that baleen filtration is not so simple as customarily presumed. This is particularly true for rorquals, where an enormous mouthful of water is rapidly (within 10-40 seconds, depending on the species) filtered following a lunge engulfment (Goldbogen, 2010; Goldbogen et al., 2017b; Shadwick et al., 2019). During this rapid process, the flow volume rate and speed of water expulsion are expected to decrease—though precisely how they decrease (for example, linearly or logarithmically) is unknown. Further, there is increasing evidence (Werth et al., 2019b) that the direction of water flow changes as rorqual filtration proceeds.
Specifically, images of asymmetrical VGB contraction and oral pouch compression (Shadwick et al., 2013), along with flow of water and bubble streams indicating the direction and degree of water exiting the mouth (e.g., Figure 8), indicate that flow through baleen occurs in discrete yet characteristic steps or stages. This likely occurs for more effective filtration leading to better capture, collection, and accumulation of prey to be swallowed (Werth and Ito, 2017). In the presumed first phase, initial gape closure appears to cause water expulsion along the full margins of the jaws. This seems to be followed by purging of a distinct stream of water just below the eye at the angle of the mouth—the same place where water steadily exits the posterior of the mouth during continuous filtration in balaenids (Werth, 2004). An apparent third and final stage involves a larger burst of purged water, again from the posterior-most angle of the mouth (Figure 9); this seemingly coincides with hyolingual movements that may correspond to swallowing of accumulated prey (Werth, 2009; Werth and Ito, 2017).
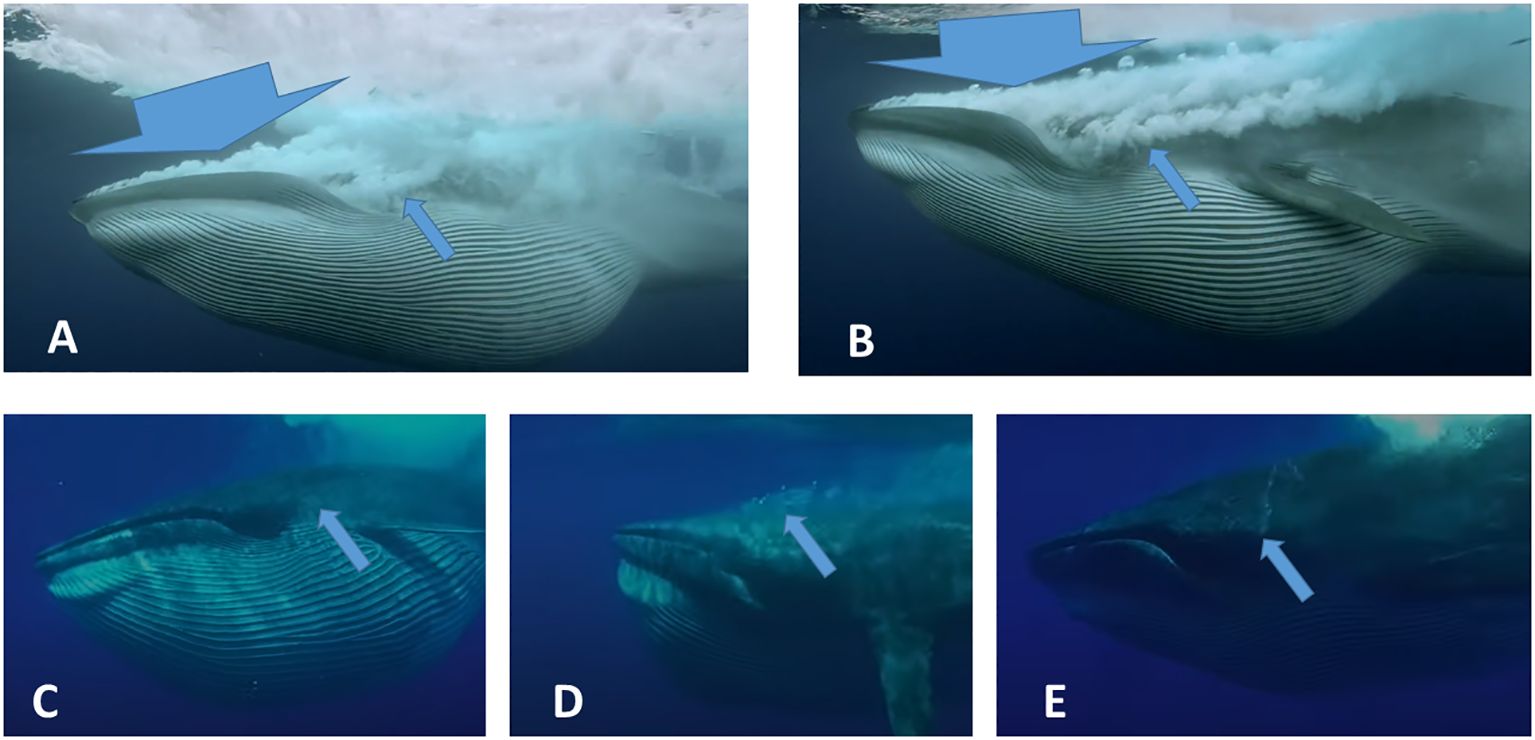
Figure 9 Sequential stages of filtration in a Bryde’s whale, Balaenoptera edeni, reflected here by the uneven, asymmetrical closure of the grooved ventral oral pouch and differential excurrent flow along the jaw margins and angle of the mouth below the eye, indicate different phases of the filtration process, presumably with varied flow directions, speeds, and prey accumulation. Large arrows in (A, B) indicate large-scale water expulsion along the entire margin of the jaw; smaller arrows indicate path of water from angle of mouth, which alone exist in later stages (C–E) after most water has been expelled. Freeze-frame images from Bob Morgan and BBC The Hunt video, courtesy Hugh Pearson.
The recognition of apparent discrete phases of mysticete filtration is a new finding that is the subject of ongoing data collection and analysis. In any case, the obvious variation between water flows at different times of whale feeding means not only that filtration is more complicated than conventionally presumed, but also that it seems to involve precisely stereotyped events that can be documented and studied, and which seem to correlate with different flows.
Hydrodynamically, what this apparent division of rorqual filtration into discrete stages means is that water likely flows in varied directions, with apparently different flow speeds and volume flow rates, as outlined in the previous two sections. There is a strong likelihood that this is related to setting up tangential flow for better, more efficient filtration. However, this is far from uncertain. Just as the previous section on tangential filtration plainly applies to balaenids but may also apply to rorquals, so too this section on discrete filtration stages applies to rorquals but may also apply to other (gray and balaenid) whales, even if less markedly.
7 Baleen’s biomechanical properties vary by location and conditions
Another largely unappreciated aspect of the baleen filter’s biomechanical complexity is related to material properties (primarily stiffness) of the metafilter’s combined components. Much more must be learned about how baleen’s diverse keratinous elements (i.e., cortical plate, tubular, and intertubular matrix keratin) grow, mature, and interact together (Werth et al., 2020), and how they are altered by inorganic material content such as calcification (Szewciw et al., 2010). The extent to which these factors directly affect plate stiffness or flexibility is gradually being recognized (Werth et al., 2016a, Werth et al., 2018b; Werth and Whaley, 2019) but remains largely unstudied.
Baleen has long been known to be strong and resilient yet pliant (Werth et al., 2018b). It is capable of withstanding strong compressive, tensile, and shear forces (Werth, 2013, Werth, 2019). Calcification stiffens baleen into the hardest alpha keratin tissue (Pautard, 1963; Szewciw et al., 2010); it is much more pliable when hydrated (Werth et al., 2016a). As a tough yet flexible tissue that does not degrade, baleen has long been used by indigenous cultures for artwork, baskets, implements, armor, and weapons (Moffat et al., 2008; Dubner, 2023). During the era of industrial whaling baleen became a highly valuable commodity, peaking in 1853 with over 5.6 million pounds, mostly from right whales, sold in U.S. ports for almost $2,000,000 (Stevenson, 1907). Fringes were made into brushes; plates were used for corset stays, skirt hoops, umbrella ribs, buggy whips, and numerous other items (Lee, 1998). Prior to the invention of plastic polymers, baleen served as the plastic of its day, such that whaling ports were among the world’s most commercially productive locales. Together with whale oil, baleen made whaling the first true American industry (Dubner, 2023).
Baleen’s commodity value derived in large part from its exceptional flexibility; its biological value for filtering also arises from this flexibility (Stevenson, 1907). Baleen plates bend along their entire length (Figure 10) in response to the forces acting upon them during filtration (Werth et al., 2018b), which enables baleen to withstand strong forces without cracking, providing great longevity. Three-point bending tests of dried and hydrated baleen specimens using an Instron E1000 ElectroPuls or Mark-10 ES30 universal testing machine enabled calculation of uniaxial flexural stress, strain, and modulus in samples of baleen plates and fringes from different whale species and different locations within a rack and plate (Figure 11). This also enabled creation of a loading (force-deformation) curve (Figure 12), with determination of elastic stiffness and yield and failure points (McKittrick et al., 2012; Werth et al., 2018b). Such experiments showed that baleen can withstand forces >1 kN without breaking (Werth et al., 2018b), and once again at levels that vary along a rack (Figure 11).
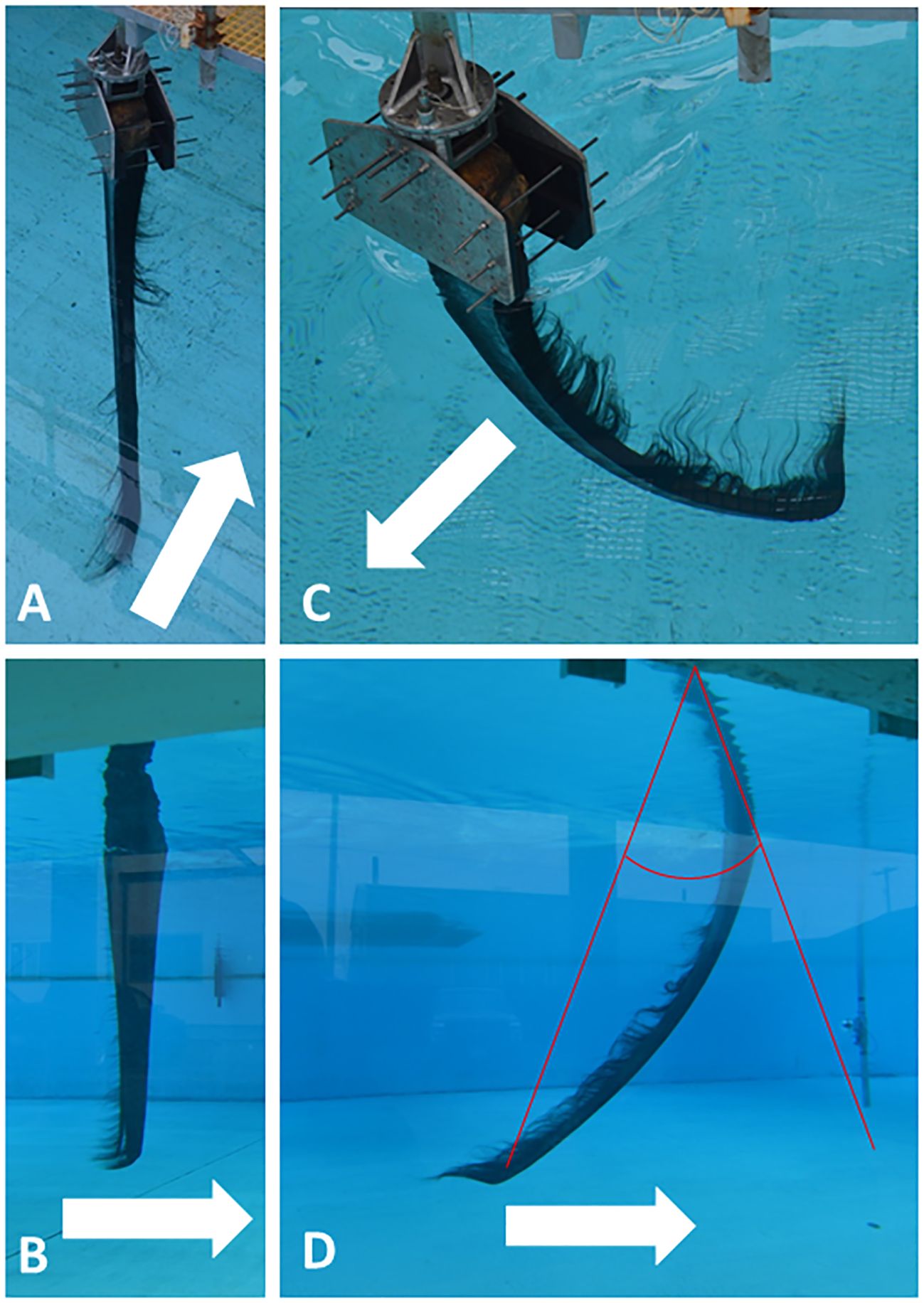
Figure 10 Tow tank experiments with “mini-racks” (30 adjacent full baleen plates of a bowhead whale, Balaena mysticetus) towed in arrow direction at 0.4 m/s (seen from surface in A and from underwater viewing window in B) and 1.2 m/s (seen from surface in C, underwater in D). Images show that baleen plates generally bend differentially along their full length due to dorsal attachment and free ventral vertex, plus varied histology/geometry by length. D also shows how plate tip bending angle was measured from photographs via divergence from the original straight axis.
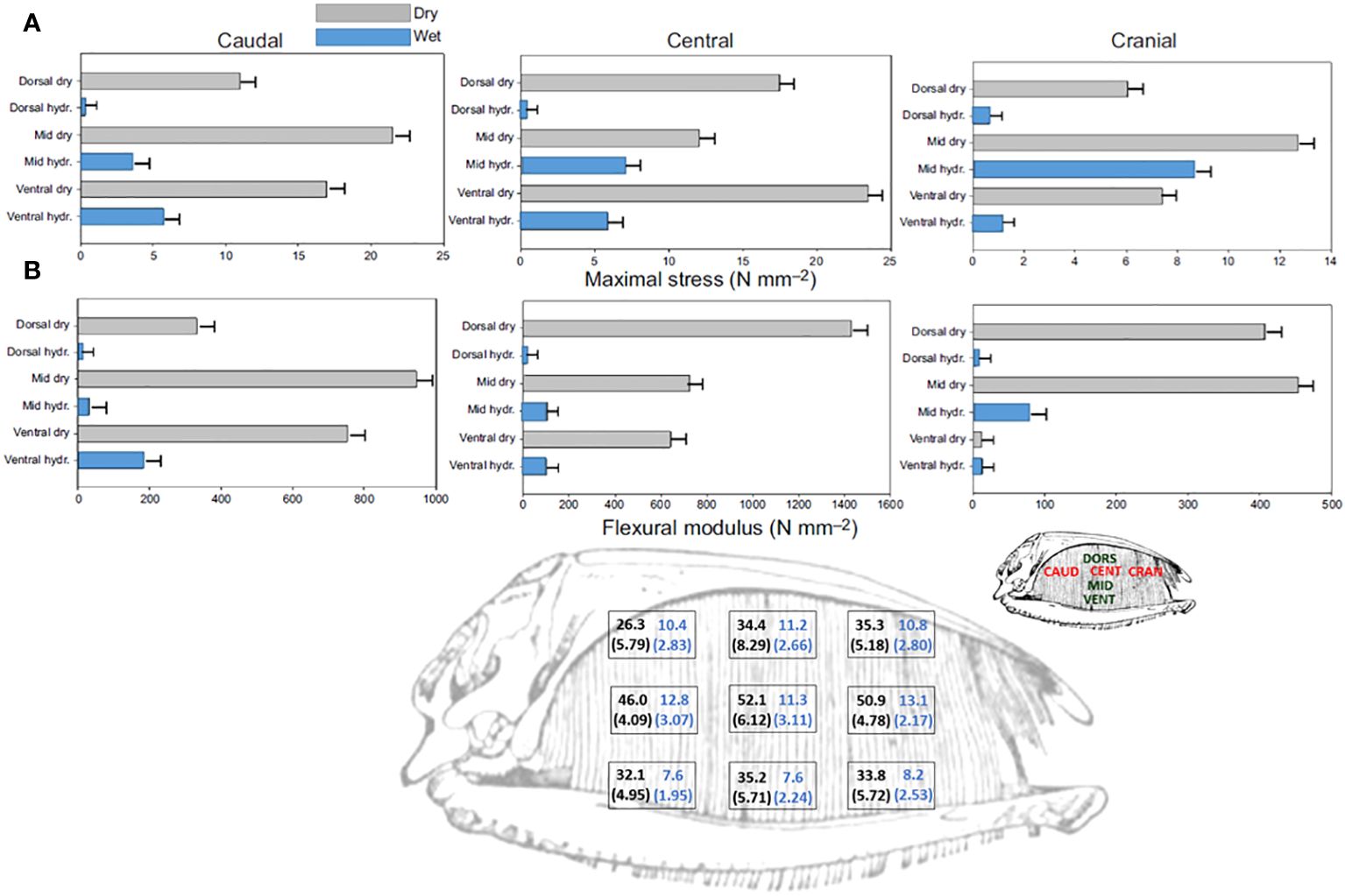
Figure 11 Maximal stress (A) and flexural stiffness (B) along baleen plates, from material strength testing of air-dried (gray bars) vs. hydrated (blue bars) right whale (Eubalaena glacialis) samples, showing data from nine locations along anteroposterior and dorsoventral axes (Werth et al., 2018b). Bottom illustration of full right whale baleen rack shows manual bending results, with mean and SD in parentheses, showing force in N from 20 experimental trials to produce 45-degree axial bending in air-dried and hydrated (black and blue numbers, respectively) right whale plates, indicating differences by location in a rack (Werth et al., 2016a; Werth et al., 2018b).
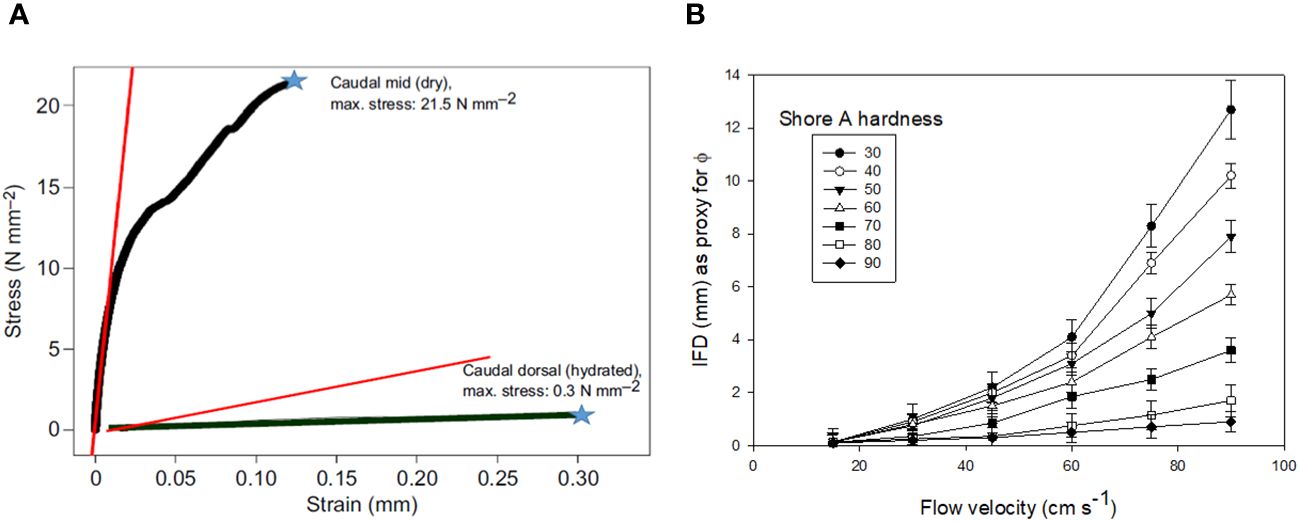
Figure 12 Mechanical testing results (A) showing maximal stress recorded from three-point bending tests of right whale baleen, showing noodle-like flexibility of wet baleen and brittle fracturing of dried baleen, which failed at 12 mm displacement (strain). (B) shows how porosity, as indicated by interfringe distance (IFD), varies by flow speed and Shore hardness, an industrial measure of rubber material softness (measured here in variably hydrated baleen plates) Data from Werth, 2013 and Werth et al., 2016a; Werth et al., 2018b, Werth et al., 2020.
These experiments (Werth, 2013; Werth et al., 2016a; Werth et al., 2018b) also demonstrated the importance of studying baleen under natural conditions—namely, when baleen is fully saturated with water, rather than dried out, the way it is typically stored and displayed in museum collections and exhibits (Werth et al., 2019c). Baleen in water—the way it perpetually is in vivo—itself absorbs and holds much water: 32.35% by weight (Werth et al., 2016a). Mechanical materials testing confirmed that dried baleen is stiff but readily shatters, whereas hydrated baleen (like other hydrated keratins; Kitchener and Vincent, 1987; Feughelman, 1997; Taylor et al., 2004) is much less stiff and bends with little applied force, yet rarely breaks (Werth et al., 2016a; Werth et al., 2018b). This is similar to the difference between dried and fully hydrated pasta noodles, and, further, demonstrates the obvious importance of studying biological materials under natural conditions. Contact angle and wettability tests (Werth et al., 2016a; Werth et al., 2019a) also confirmed that hydrated baleen is highly hydrophilic and oleophobic (i.e., oil-shedding), which is important given that small schooling fish and zooplankton have bodies filled with oil droplets to store energy and maintain neutral buoyancy (St. Aubin et al., 1984; Michaud and Taggart, 2007).
Baleen plates of bowhead and right whales are so long (potentially 4+ m long in large adults) that they must bend to be stowed when the mouth is closed (Werth et al., 2018b). This raises the question of how and where plates bend: uniformly along their length (Figure 10), or are they more “hinged” where they emerge from the dorsal gingiva? Plates were materially tested for differential stiffness, using three-point bending tests, along two axes with a full baleen rack: anteroposterior and dorsoventral. Results indicate that plates are more stiff in the central portion (Figure 12), midway between the newest baleen that emerges from the palate and oldest baleen at the ventral apex of the plate. The baleen at the “top” of each plate is newest and least worn (hence thickest and widest, with fewer free fringes), and it is also stably fixed to the palate; the oldest baleen at the ventral vertex is most eroded (thin and narrow, with many free fringes) and it is furthest from its fixed attachment point. These factors—anatomical, geometric, life history/developmental, etc.—all play crucial roles in the structure and function of the keratinous oral filter. Mechanical testing of baleen from rorquals (blue, fin, humpback, and minke whales; Werth et al., 2018b) indicates similar material properties to balaenid baleen, but markedly less flexibility and fewer dorsoventral differences along the shorter rorqual plates. Baleen fringes from all species are highly flexible and elastically ductile along their length; they bend easily but resume their original form. Thus fringes are not easily broken, but readily they separate from each other to form the fibrous mat portion of the metafilter, to yield rack location-dependent porosities and hydrodynamic performance (Figure 4; Werth et al., 2018a; Potvin and Werth, 2024).
Histological examination reveals that the internal hollow horn tubules which eventually emerge as eroded fringes provide another key way in which baleen plates differ along their axes (Figure 13). In both bowhead and fin whales, tubule density is significantly greater on the medial (lingual) side of plates relative to the lateral (labial) side, as revealed by comparative histological study (Werth et al., 2018b). This abundance of medial fringes likely helps to stiffen and resist fracture on the side of the filter that sees the most “action” from intense water flow, prey accumulation, and possible interaction with the tongue (discussed in the next section). Moreover, the profusion of medial tubules also leads directly to the high density of abundant eroded fringes on the medial or incurrent “business” side of the filter. Modern imaging techniques such as scanning and atomic force microscopy (Figure 14) are helping to resolve the microscopic ultrastructure of the three-dimensional complexity tissue underlying the baleen metafilter (Werth et al., 2018a, Werth et al., 2018b). Our view of the ways in which baleen’s ultrastructure influence and determine its porosity, stiffness, and other functional properties is slowly resolving, with much more work to be done.
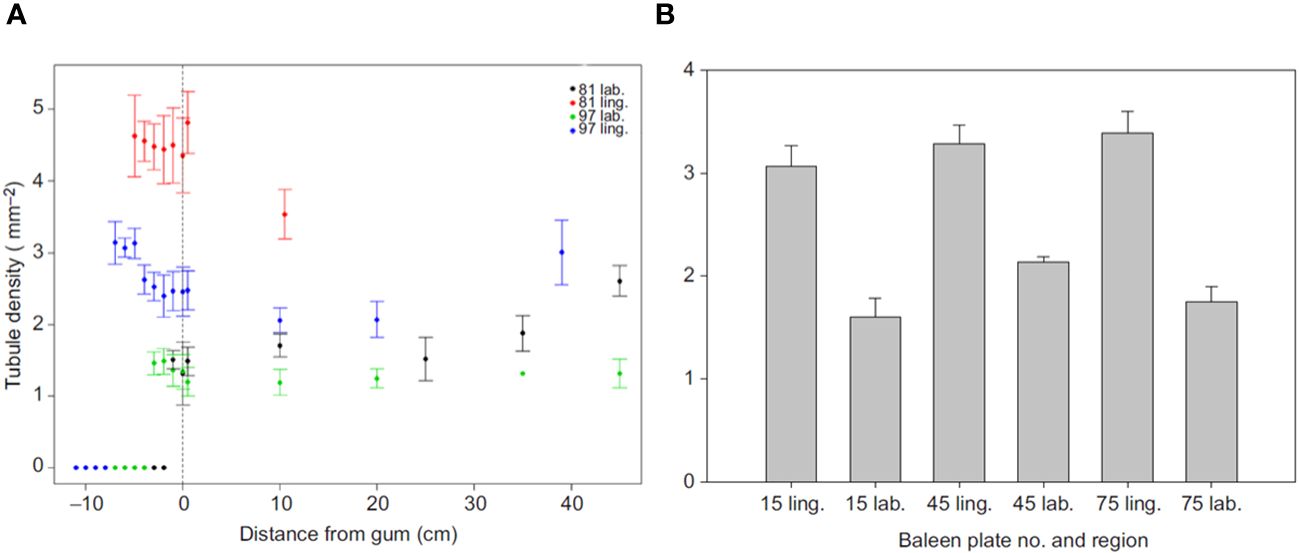
Figure 13 Comparative density of hollow horn tubules (mean+SD) in two fin whale baleen plates (A) and three bowhead whale plates (B) shows greater tubule density in the medial (inner or lingual) plate region relative to the lateral (outer, labial) region in both species. Images revised from Werth et al., 2018b.
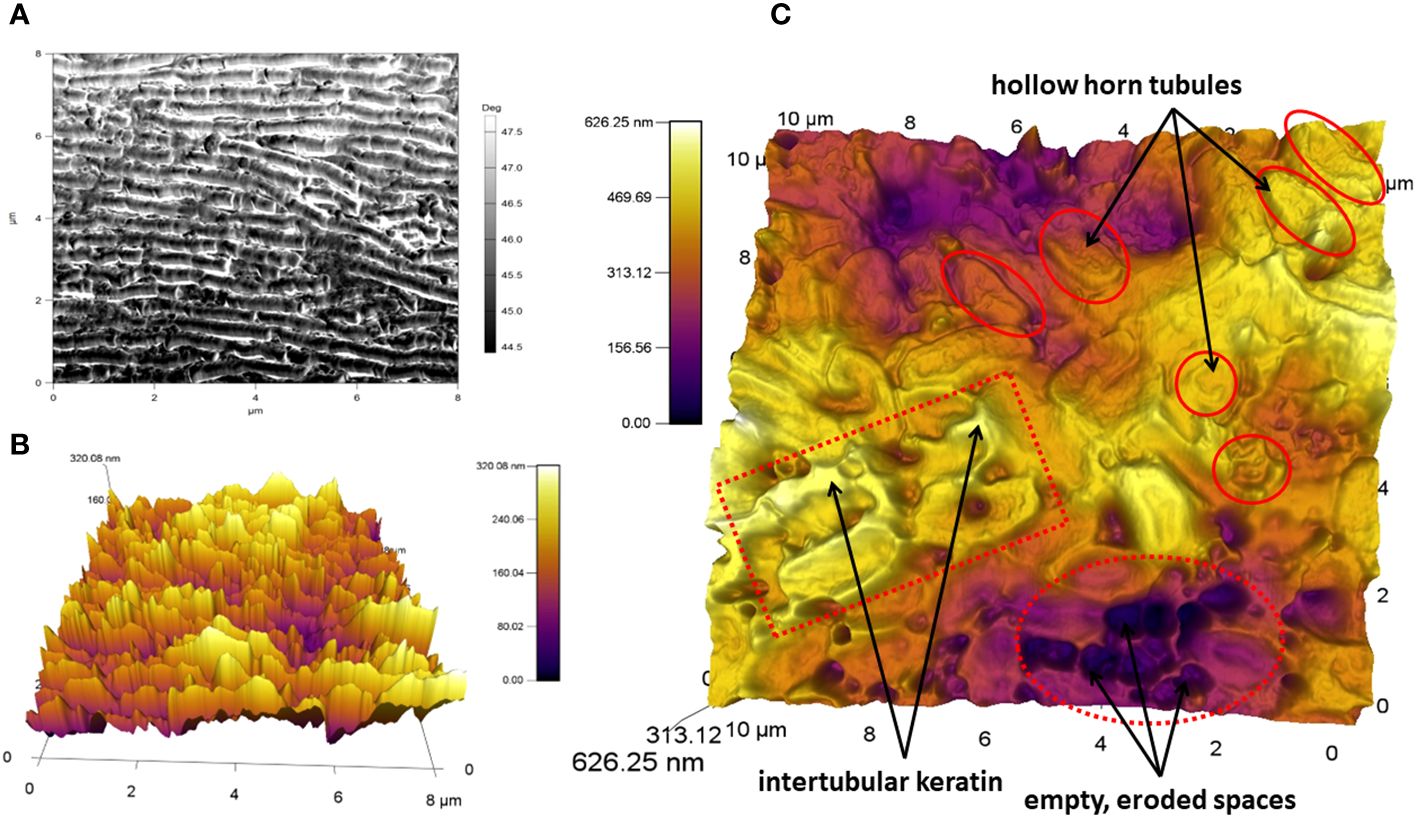
Figure 14 Atomic force microscopy (AFM) image of a bowhead baleen sample reveal relative smoothness of plate surface with underlying periodicity of growth lines (A), and topographic 3D rendering of baleen’s ultrastructural material complexity (B, C) related to diverse keratinous components plus spaces where plates erode and tubules separate. Images revised from Werth et al., 2020.
8 Variable means to clear or clean retentate from baleen filter
A strong benefit of using cross-flow or other tangential filtration during mysticete feeding would be better clearing of the filter, with lesser accumulation of solids directly upon the baleen rack and instead greater accumulation mostly posterior to it. Bulk deposition of prey toward or at the rear of the mouth (by the tongue root) would not only aid swallowing, but also minimize or preclude filter clogging. If water flows mainly along rather than straight through the fringe mat and inter-plate gaps, prey items cannot easily become stuck within the baleen metafilter. As mentioned earlier, the slowed flow through and along the filter’s posterior section, along with the accumulated bolus or slurry of prey (with reduced water content) would also facilitate swallowing of prey without swallowing of unnecessary water.
But even if individual or bulk prey were to become enmeshed within the filter, how could they be removed? If baleen were to become clogged, how might it be unclogged? Werth (2001) envisioned three distinct scenarios whereby prey trapped in the baleen filter could be removed. The simplest and most obvious solution involves direct mechanical scraping or shaking of the filter by the tongue (Figure 15). Although this might work, there are several potential problems. First, this overestimates the mobility and muscularity of the tongue body in adult rorquals, which, in order to invaginate and line the ventral cavity of the throat pouch during lunge engulfment (Lambertsen, 1983), resembles a floppy, flaccid waterbed more than a firm tongue akin to that of most mammals (Werth and Crompton, 2023). Second, even if the tongue were not too loose to dislodge ensnared items, prey might be pushed even deeper into the filter mat, compounding the problem. Third, this mechanical abrasion might cause baleen to erode faster than it can be replaced by new growth (Werth et al., 2020). Using the tongue to shake the filtering baleen plates and fringes might work better than directly scraping them, and there is some evidence of a “baleen rattle” of adjacent plates clacking together, although this is generally heard during surface skimming in balaenids when gape is open (Watkins and Schevill, 1976), not when gape closes for presumed filter clearing and swallowing (Werth, 2001).
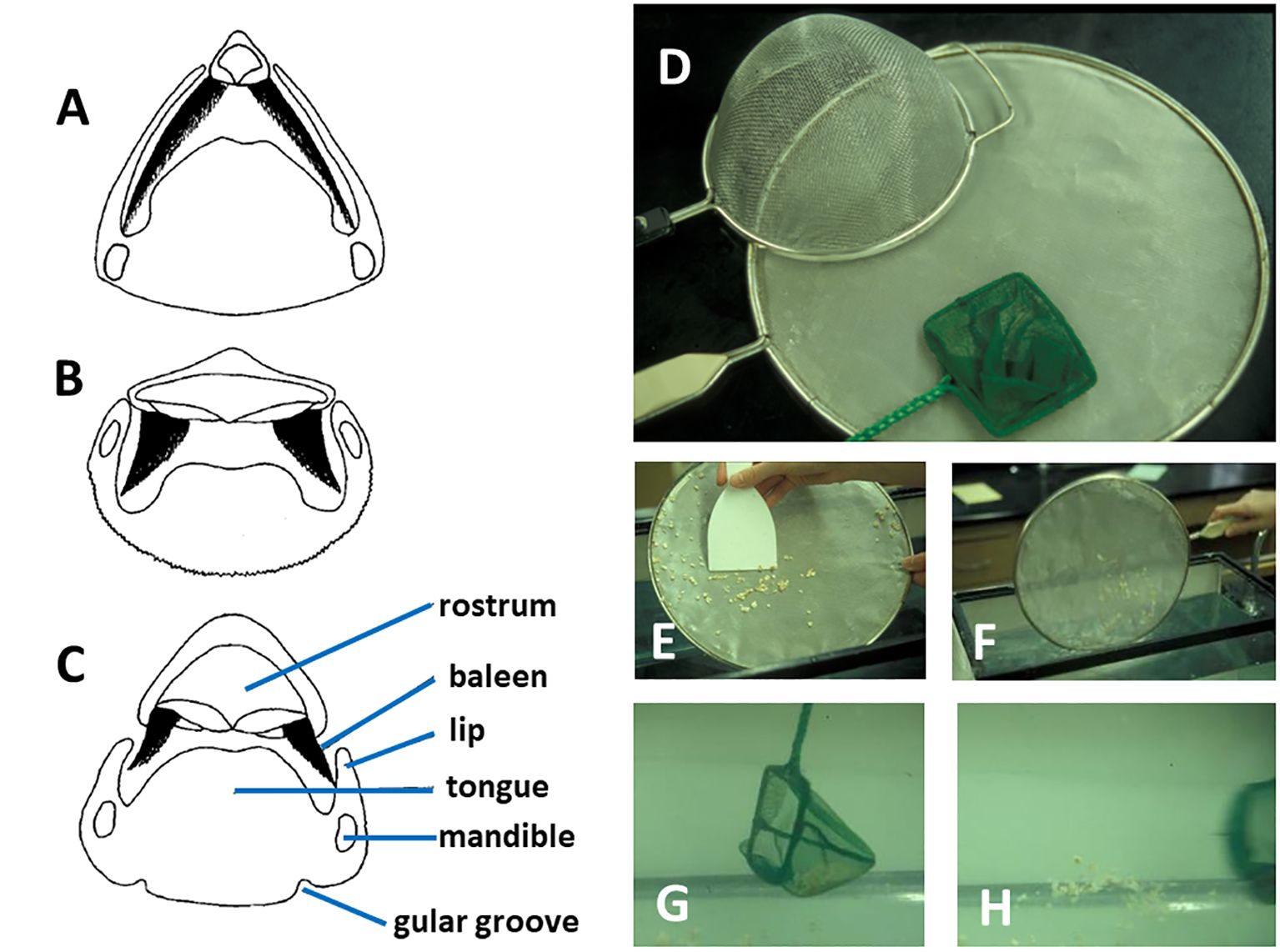
Figure 15 Transverse sections of whale heads/mouths ((A) balaenid, (B) rorqual, (C) gray whale) as depicted in Figure 2 show potential physical interactions of dark triangular baleen and other oral structures, which may relate to cleaning/clearing of prey from baleen. This is analogous to three options to clean a clogged filter or dipnet (D) by mechanical scraping (E), physical shaking (F), or backwash flushing of the filter (G, H).
A second scenario to remove prey trapped in the baleen filter would be to rapidly shake or nod the head, with loosened items falling to the tongue assisted by gravity and inertia (Figure 9). Some field observations, especially of right whales, appear to support claims of head shaking or “nodding” behavior (Gaskin, 1982; Werth, 2001). Although this might provide sufficient force to dislodge items from a clogged filter, it would be difficult to achieve, as head motion would necessitate major expense of metabolic energy. It would also disrupt forward locomotion, which seems not to be impeded during mysticete filtration (beyond typical drag forces) as evidenced by aerial drone recordings (Werth et al., 2019b) and by accelerometers and other instruments from attached tags (Goldbogen et al., 2017a).
A third scenario whereby mysticetes might clear their filter of trapped items would use hydraulic or hydrodynamic rather than brute mechanical forces. Rapid depression and retraction of the tongue, as used to generate intraoral suction for prey ingestion in gray whales (Ray and Schevill, 1974), could create a rapid “backwash” flow to flush and dislodge trapped prey (Figure 15). This could be readily achieved with relatively low metabolic cost and without impeding locomotion or damaging baleen. However, many prey items, both fish and zooplankton, and negatively rheotropic—swimming away from the direction of current flow—so they might burrow deeper into the baleen filter if a backwash current were insufficiently strong to pull them out of the filter (Werth, 2001). Another hydraulic solution could involve the intraoral vortical flows described earlier, which could help to clean baleen fringe mats or indeed to keep them clear from clogging in the first place. To the extent that any tangential flow is involved, it would of course minimize the need to free trapped prey.
All three of these (and other as yet undescribed) possibilities could apply, and they are not mutually exclusive. The best solution might be some combination, or varied mechanisms might be used by different whales in different situations. The main conclusions are that oral filter clogging remains a real even if remote possibility, and that this provides yet another aspect of baleen feeding’s multifaceted complexity. Future investigations of this topic should focus on laboratory experiments demonstrating potential baleen clogging and clearing, morphological and prey data from baleen racks studied during necropsy examination, and field observations of whale behavior (e.g., head nodding, shaking, or backwash flow) potentially relating to cleaning of the baleen filter.
9 Conclusions and future plans
Over the past two decades, novel approaches and techniques such as biologging (Goldbogen et al., 2017a) and UAV videography (Werth et al., 2019b) have greatly expanded the number of structural and functional studies on baleen, and have similarly expanded our scientific understanding of baleen’s role in filter feeding. Chief among these, as described in the preceding sections, are the recognition of cross-flow filtration, porosity that varies by flow parameters, differing roles for baleen plates and fringes, and biomechanical properties that vary by location within the overall baleen metafilter. We now understand that mysticete filtration does not depend solely on simple sieving. That is the good news. Unfortunately, new questions appear with every answer these new studies reveal, such as how whales locate food over different scales of distance, and how they control and know precisely when to open and close the mouth. Other aspects of whale filtration demanding further study include intraoral pressure gradients and fluid geometry (i.e., how water flow changes direction) as the mouth fills and empties during feeding.
In trading our conventional view of baleen as a static sieve with our newfound appreciation of baleen as a highly complex and dynamically variable filter, we are gaining a more realistic understanding of how whales feed, but at the expense of short, simple conceptual explanations. The more we understand the morphology and fluid dynamics of baleen filtration, the better we can protect whales from crucial environmental risks such as ingestion of plastic debris (Werth et al., 2024) or entanglement of baleen in fishing gear (van der Hoop et al., 2015; Lysiak et al., 2018). For example, determining precisely how water flows into and out of whale mouths could potentially mitigate harm from entangling ropes that bend or otherwise damage baleen plates, disrupting filtration. This could also help to foster strategies for developing fishing lines that detach in ways suited to mysticetes’ oral anatomy and flow regimes. Improved knowledge of mysticete filtration could also lower risks posed by whales’ ingestion of oil or plastic debris
Future research efforts exploring whale filtration should benefit from advances in UAV “drone” and biologging (i.e., archival and telemetric tag) technology, and in computational fluid dynamics and modeling. Flume experiments should continue to study details of water flow vectors, speeds, timing, and so on. The possibility of recording data from inside a whale mouth, with swallowed, anchored, or “walking” tags, has been a tantalizing dream of scientists. Data from all such investigations would be valuable in addressing gaps in current knowledge, particularly regarding flow parameters, and in connecting the physics of flow with the ecology of living whales, which are remarkably difficult to study due to obvious logistical, legal, and ethical considerations.Biomimetics—the application of biologically inspired designs to build better structures and materials for human use (Trakumas et al., 2001; Cohen et al., 2014)—can apply many useful findings from recent and ongoing baleen research. Specifically, baleen’s composite keratinous material, the geometry of the mysticete oral metafilter and its fluid dynamics, the precise mechanisms of particle flow and collection, the pathways of water entry and effluence, the forces and pressure gradients that baleen encounters during filtration, and methods of clearing the filter are all key points that could be exploited via biomimetics. Bioinspired structures and systems based on improved knowledge of baleen function could have many conceivable applications that would prove valuable for a range of technical and industrial design concerns, such as how to improve filter flow and efficiency while limiting breakage and clogging, and how to deal with real-world environmental issues such as clearing waters of microplastics and other waste (Hung et al., 2012; Zhong et al., 2012; Bach et al., 2015; Divi et al., 2018; Schroeder et al., 2019; Witkop et al., 2023).
The other real benefit of our deeper, more genuine understanding of baleen filtration comes from potentially broader application to multiple filtration scenarios, both in biology and in applied industrial settings. Together, all the findings of the past two decades highlighted in each of the sections above have not only greatly elucidated processes of baleen function but also heightened the need for further research. Many aspects of baleen filtration probably pertain to other biological filters, and can better enable engineers and industrial designers to create artificial filters that are more efficient and longer-lasting.
This is a two-way street, however. One can experiment on or otherwise use knowledge of man-made filters with varying stiffness or other material properties (Mironov et al., 2003; Yeong et al., 2004) to better understand exactly how baleen works in vivo in actual whale mouths, something that is still not fully understood. The many prominent hurdles that limit research on whales (legal, logistical, and fiscal) are impediments to carrying out many basic experiments beyond observing structures in dead whales. Fortunately, testing of 3-D printed models of whale baleen (Mironov et al., 2003; Jensen et al., 2017), especially those with exaggerated, unrealistic stiffness or other material properties, can show us how nature does and does not work, better revealing why baleen looks and acts the way it does (Werth, 2019). This reversal of conventional biomimetics—in which, rather than nature inspiring novel technologies, the new technological applications and findings instead further inform our understanding of nature—holds great promise. In this way we can better understand natural systems, allowing us to design and manufacture more effective synthetic filters. Perhaps most importantly and fruitfully, research must continue to study the numerous important ways in which baleen offers a highly dynamic and variable filtering paradigm. Understanding common versus unique features of varied filtration phenomena, both biological and artificial, will continue to aid scientific and technical understanding, enable fruitful interdisciplinary partnerships, and yield new filter designs.
Author contributions
AW: Conceptualization, Supervision, Writing – original draft, Writing – review & editing. JP: Conceptualization, Supervision, Writing – review & editing.
Funding
The author(s) declare that no financial support was received for the research, authorship, and/or publication of this article.
Acknowledgments
The authors acknowledge dozens of research colleagues (including those not specifically listed here), most of whom have been collaborative investigators and all of whom have provided valuable ideas and feedback. Especially helpful collaborators and colleagues include Jeremy Goldbogen, Bob Shadwick, Nick Pyenson, Haruka Ito, Michael Moore, Brian Kot, Peter Madsen, Peter van de Graaf, Margo Lillie, Shirel Kahane-Rapport, Dave Cade, Matt Savoca, Wayne Vogl, Ewan Fordyce, Jan Straley, Tom Ford, Joy Reidenberg, and Ann Pabst. The authors also thank the editor and reviewers for helpful comments and suggestions.
Conflict of interest
The authors declare that the research was conducted in the absence of any commercial or financial relationships that could be construed as a potential conflict of interest.
Publisher’s note
All claims expressed in this article are solely those of the authors and do not necessarily represent those of their affiliated organizations, or those of the publisher, the editors and the reviewers. Any product that may be evaluated in this article, or claim that may be made by its manufacturer, is not guaranteed or endorsed by the publisher.
References
Bach D., Schmich F., Masselter T., Speck T. (2015). A review of selected pumping systems in nature and engineering—potential biomimetic concepts for improving displacement pumps and pulsation damping. Bioinspiration Biomimetics 10, 051001. doi: 10.1088/1748-3190/10/5/051001
Baker R. J., Fane A. G., Fell C. J. D., Yoo B. H. (1985). Factors affecting flux in crossflow filtration. Desalination 53, 81–93. doi: 10.1016/0011-9164(85)85053-0
Bannister J. L. (2008). Baleen whales, in Encyclopedia of Marine Mammals 3e. Eds. Würsig B., Thewissen J. G. M., Kovacs K. (New York: Elsevier), pp 80–pp 89.
Belfort D., Davis R. H., Zydney A. L. (1994). The behavior of suspensions and macromolecular solutions in crossflow microfiltration. J. Membrane Sci. 96, 1–58. doi: 10.1016/0376-7388(94)00119-7
Berta A., Lanzetti A., Ekdale E. G., Deméré T. A. (2016). From teeth to baleen and raptorial to bulk filter feeding in mysticete cetaceans: the role of paleontological, genetic, and geochemical data in feeding evolution and ecology. Integr. Comp. Biol. 56, 1271–1284. doi: 10.1093/icb/icw128
Berta A., Sumich J. L., Kovacs K. (2015). Marine Mammals: Evolutionary Biology 3e (San Diego: Elsevier).
Bisconti M. (2012). Comparative osteology and phylogenetic relationships of Miocaperea pulchra, the first fossil pygmy right whale genus and species (Cetacea, Mysticeti, Neobalaenidae). Zoological J. Linn. Soc. 166, 876–911. doi: 10.1111/zoj.2012.166.issue-4
Bisconti M., Pellegrino L., Carnevale G. (2021). Evolution of gigantism in right and bowhead whales (Cetacea: Mysticeti: Balaenidae). Biol. J. Linn. Soc. 134, 498–524. doi: 10.1093/biolinnean/blab086
Bisconti M., Pellegrino L., Carnevale G. (2023). The chronology of mysticete diversification (Mammalia, Cetacea, Mysticeti): body size, morphological evolution and global change. Earth Sci. Rev. 239, e104373. doi: 10.1016/j.earscirev.2023.104373
Bisconti M., Varola A. (2006). The oldest eschrichtiid mysticete and a new morphological diagnosis of Eschrichtiidae (gray whales). Rev. Italiana di Paleontologia e Stratigraphia 112, 447–457. doi: 10.13130/2039-4942/6352
Blatt W., Dravid A., Michael A. S., Nelson L. (1970). Solute polarization and cake formation in membrane ultrafiltration: causes, consequences and control techniques, in Membrane Science and Technology. Ed. Flinn J. E. (New York: Plenum), pp 47–pp 91.
Boessenecker R. W., Fordyce R. E. (2015). Anatomy, feeding ecology, and ontogeny of a transitional baleen whale: a new genus and species of Eomysticetidae (Mammalia: Cetacea) from the Oligocene of New Zealand. PeerJ 3, 1129. doi: 10.7717/peerj.1129
Bosio G., Collareta A., DiCelma C., Lambert O., Marx F. G., de Muizon C., et al. (2021). Taphonomy of marine vertebrates of the Pisco Formation (Miocene, Peru): insights into the origin of an outstanding fossil-lagerstätte. PLoS One 16, e0254395. doi: 10.1371/journal.pone.0254395
Bott R., Langeloh T. H., Ehrfeld E. (2000). Dynamic cross flow filtration. Chem. Eng. J. 80, 245–249. doi: 10.1016/S1383-5866(00)00097-6
Brodie P., Vikingsson G. (2009). On the feeding mechanisms of the sei whale (Balaenoptera borealis). J. Northwest Atlantic Fisheries Sci. 42, 49–54. doi: 10.2960/J.v42.m646
Cade D. E., Friedlaender A. S., Calambokidis J., Goldbogen J. A. (2016). Kinematic diversity in rorqual whale feeding mechanisms. Curr. Biol. 26, 2617–2624. doi: 10.1016/j.cub.2016.07.037
Caraveo-Patiño J., Hobson K. A., Soto L. A. (2007). Feeding ecology of gray whales inferred from stable-carbon and nitrogen isotopic analysis of baleen plates. Hydrobiology 586, 17–25. doi: 10.1007/s10750-006-0477-5
Cheer A., Cheung S., Hung T.-C., Piedrahita R. H., Sanderson S. L. (2012). Computational fluid dynamics of fish gill rakers during crossflow filtration. Bull. Math. Biol. 74, 981–1000. doi: 10.1007/s11538-011-9709-6
Cheer A. Y., Ogami Y., Sanderson S. L. (2001). Computational fluid dynamics in the oral cavity of ram suspension-feeding fishes. J. Theor. Biol. 210, 463–474. doi: 10.1006/jtbi.2001.2325
Cohen Y. H., Reich Y., Greenberg S. (2014). Biomimetics: Structure–function patterns approach. J. Mechanical Design 136, 111108–111101. doi: 10.1115/1.4028169
Collareta A., Gioncada A., Gariboldi K., Bonnacorsi A., Marx F. G., Lambert O., et al. (2017). Fossil baleen under the microscope: seeking the key for fine-scale preservation of soft tissues, in Geosciences: a tool in a changing world [abstract book] (Pisa, Italy: Società Italiana di Mineralogia e Petrografia), p 255.
Collareta A., Landini W., Lambert O., Post K., Tinelli C., DiCelma C., et al. (2015). Piscivory in a Miocene Cetotheriidae of Peru: first record of fossilized stomach content for an extinct baleen-bearing whale. Sci. Nat. 102, e00114–015-1319. doi: 10.1007/s00114-015-1319-y
Croll D. A., Tershy B. R., Newton K. M. (2008). Filter feeding, in Encyclopedia of Marine Mammals 3e. Eds. Würsig B., Thewissen J. G. M., Kovacs K. (New York: Elsevier), pp 429–pp 433.
Deméré T. A., McGowen M. R., Berta A., Gatesy J. (2008). Morphological and molecular evidence for a stepwise evolutionary transition from teeth to baleen in mysticete whales. Systematic Biol. 57, 15–37. doi: 10.1080/10635150701884632
Divi R. V., Strother J. A., Paig-Tran E. W. M. (2018). Manta rays feed using ricochet separation, a novel nonclogging filtration mechanism. Sci. Adv. 4, e9533. doi: 10.1126/sciadv.aat9533
Dubner S. (2023) The first great American industry (Freakonomics). Available online at: https://freakonomics.com/podcast/the-first-great-american-industry/ (Accessed 12 July 2023).
Ekdale E. G., Deméré T. A. (2021). Neurovascular evidence for a co-occurrence of teeth and baleen in an Oligocene mysticete and the transition to filter-feeding in baleen whales. Zoological J. Linn. Soc. 194, 395–415. doi: 10.1093/zoolinnean/zlab017
El Adli J. J., Deméré T. A., Boessenecker R. W. (2014). Herpetocetus morrowi (Cetacea: Mysticeti), a new diminutive baleen whales from the Upper Pliocene (Piacenzian) of California, USA, with observations on the evolution and relationships of the Cetotheriidae. Zoological J. Linn. Soc. 170, 400–466. doi: 10.1111/zoj.2014.170.issue-2
Esperante R., Brand L., Nick K. E., Poma O., Urbina M. (2008). Exceptional occurrence of fossil baleen in shallow marine sediments of the Neogene Pisco Formation, Southern Peru. Palaeogeography Palaeoclimatology Palaeoecol. 257, 344–360. doi: 10.1016/j.palaeo.2007.11.001
Espinosa-Gayosso A., Ghisalberti M., Ivey G. N., Jones N. L. (2012). Particle capture and low-Reynolds-number flow around a circular cylinder. J. Fluid Mechanics 710, 362–378. doi: 10.1017/jfm.2012.367
Feughelman M. (1997). The relation between the mechanical properties of wool and hair fibres and the keratin-water system, in Mechanical Properties and Structure of Alpha-Keratin Fibres. Ed. Feughelman M. (Sydney: Univ. of New South Wales Press), pp16–pp27.
Feughelman M. (2002). Natural protein fibers. J. Appl. Polymer Sci. 83, 489–507. doi: 10.1002/app.2255
Fields D. M., Yen J. (1997). The escape behavior of marine copepods in response to a quantifiable fluid mechanical disturbance. J. Plankton Res. 19, 1289–1304. doi: 10.1093/plankt/19.9.1289
Fitzgerald E. M. G. (2010). The morphology and systematics of Mammalodon colliveri, a toothed mysticete from the Oligocene of Australia. Zoological J. Linn. Soc. 158, 367–476. doi: 10.1111/j.1096-3642.2009.00572.x
Fordyce R. E. (1980). Whale evolution and Oligocene southern ocean environments. Palaeogeography Palaeoclimatology Palaeoecol. 31, 319–336. doi: 10.1016/0031-0182(80)90024-3
Fordyce R. E., Barnes L. G. (1994). The evolutionary history of whales and dolphins. Annu. Rev. Earth Planetary Sci. 22, 419–455. doi: 10.1146/annurev.ea.22.050194.002223
Fordyce R. E., Marx F. G. (2013). The pygmy right whale Caperea marginata: the last of the cetotheres. Proc. R. Soc. B: Biol. Sci. 280, 2645. doi: 10.1098/rspb.2012.2645
Fordyce R. E., Marx F. G. (2018). Gigantism precedes filter feeding in baleen whale evolution. Curr. Biol. 28, 1–7. doi: 10.1016/j.cub.2018.04.027
Forslind B. (1970). Biophysical studies of normal nail. Acta Dermatol. Venereology 50, 161–168. doi: 10.2340/0001555550161168
Fortune S. M. E., Trites A. W., Perryman W. L., Moore M. J., Pettis H. M., Lynn M. S. (2012). Growth and rapid early development of North Atlantic right whales (Eubalaena glacialis). J. Mammalogy 93, 1342–1354. doi: 10.1644/11-MAMM-A-297.1
Fraser R. D., MacRae T. P., Rogers G. E. (1972). Keratins: Their Composition, Structure, and Biosynthesis (Springfield IL: Charles C. Thomas).
Fraser R. D. B., MacRae T. P., Suzuki E. (1976). Structure of keratin microfibril. J. Mol. Biol. 108, 435–452. doi: 10.1016/S0022-2836(76)80129-5
Fudge D. S., Szewciw L. J., Schwalb A. N. (2009). Morphology and development of blue whale baleen: An annotated translation of Tycho Tullberg’s classic 1883 paper. Aquat. Mammals 35, 226–252. doi: 10.1578/AM.35.2.2009.226
Gatesy J., Ekdale E. G., Deméré T. A., LAnzetti A., Randall J., Beta A., et al. (2022). Anatomical, ontogenetic, and genomic homologies guide reconstructions of the teeth-to-baleen transition in mysticete whales. J. Mamm. Evol. 29, 891–930. doi: 10.1007/s10914-022-09614-8
Gatesy J., Geisler J. H., Chang J., Buell C., Berta A., Meredith R. W., et al. (2013). A phylogenetic blueprint for a modern whale. Mol. Phylogenet. Evol. 66, 479–506. doi: 10.1016/j.ympev.2012.10.012
Gatesy J., O’Leary M. A. (2001). Deciphering whale origins with molecules and fossils. Trends Ecol. Evol. 16, 562–571. doi: 10.1016/S0169-5347(01)02236-4
Geisler J. H., Boessenecker R. W., Brown M., Beatty B. L. (2017). The origin of filter feeding in whales. Curr. Biol. 2017, 2036–2042. doi: 10.1016/j.cub.2017.06.003
George J. C., Lubetkin S. C., Zeh J. E., Thewissen J. G. M., Wetzel D., Givens G. H. (2021). Age estimation, in The Bowhead Whale, Balaena mysticetus: Biology and Human Interactions. Eds. George J. C., Thewissen J. G. M. (San Diego: Academic Press), pp 309–pp 322.
George J. C., Stimmelmayr R., Suydam R., Usip S., Givens G., Sformo T., et al. (2016). Severe bone loss as part of the life history strategy of bowhead whales. PLoS One 11, e0156753. doi: 10.1371/journal.pone.0156753
Gioncada A., Collareta A., Gariboldi K., Lambert O., DiCelma C., Bonaccorsi E., et al. (2016). Inside baleen: exceptional microstructure preservation in a late Miocene whale skeleton from Peru. Geology 44, 839–842. doi: 10.1130/G38216.1
Goldbogen J. A. (2010). The ultimate mouthful: lunge-feeding in rorqual whales. Am. Scientist 98, 124–131. doi: 10.1511/2010.83.124
Goldbogen J. A., Cade D. E., Boersma A. T., Calambokidis J., Kahane-Rapport S. R., Segre P. S., et al. (2017a). Using digital tags with integrated video and inertial sensors to study moving morphology and associated function in large aquatic vertebrates. Anatomical Rec. 300, 1935–1941. doi: 10.1002/ar.23650
Goldbogen J. A., Cade D., Calambokidis J. A., Friedlaender A. S., Potvin J., Segre P. S., et al. (2017b). How baleen whales feed: the biomechanics of engulfment and filtration. Annu. Rev. Mar. Sci. 9, 367–386. doi: 10.1146/annurev-marine-122414-033905
Goldbogen J. A., Calambokidis J., Croll D., McKenna M. F., Potvin J., Pyenson N. D., et al. (2012a). Scaling of lunge feeding performance in rorqual whales: mass-specific energy expenditure increases with body size and progressively limits diving capacity. Funct. Ecol. 26, 216–226. doi: 10.1111/j.1365-2435.2011.01905.x
Goldbogen J. A., Calambokidis J., Friedlaender A. S., Francis J., DeRuiter S. L., Stimpert A. K., et al. (2012b). Underwater acrobatics by the world’s largest predator: 360 degrees rolling manoeuvres by lunge-feeding blue whales. Biol. Lett. 9, 0986. doi: 10.1098/rsbl.2012.0986
Goldbogen J. A., Calambokidis J., Oleson E., Potvin J., Pyenson N. D., Schorr G., et al. (2011). Mechanics, hydrodynamics and energetics of blue whale lunge feeding: efficiency dependence on krill density. J. Exp. Biol. 214, 131–146. doi: 10.1242/jeb.048157
Goldbogen J. A., Calambokidis J., Shadwick R. E., Oleson E., McDonald M. A., Hildebrand J. A. (2006). Kinematics of foraging dives and lunge-feeding in fin whales. J. Exp. Biol. 209, 1231–1244. doi: 10.1242/jeb.02135
Goldbogen J. A., Friedlaender A. S., Calambokidis J., McKenna M. F., Simon M., Nowacek D. P. (2013). Integrative approaches to the study of baleen whale diving behavior, feeding performance, and foraging ecology. Bioscience 63, 90–100. doi: 10.1525/bio.2013.63.2.5
Goldbogen J. A., Hazen E. L., Friedlaender A. S., Calambokidis J., DeRuiters S. L., Stimpert A. K., et al. (2015). Prey density and distribution drive the three-dimensional foraging strategies of the largest filter feeder. Funct. Ecol. 29, 951–961. doi: 10.1111/1365-2435.12395
Goldbogen J. A., Madsen P. T. (2018). The evolution of foraging capacity and gigantism in cetaceans. J. Exp. Biol. 221. doi: 10.1242/jeb.166033
Goldbogen J. A., Potvin J., Shadwick R. E. (2010). Skull and buccal cavity allometry increase mass-specific engulfment capacity in fin whales. Proc. R. Soc. B: Biol. Sci. 277, 861–868. doi: 10.1098/rspb.2009.1680
Goldbogen J. A., Pyenson N. D., Madsen P. T. (2023). How whales dive, feast, and fast: the ecophysiological limits of foraging in the evolution of Cetacea. Annu. Rev. Ecology Evolution Systematics 54, 307–325. doi: 10.1146/annurev-ecolsys-102220-025458
Goldbogen J. A., Pyenson N. D., Shadwick R. E. (2007). Big gulps require high drag for fin whale lunge feeding. Mar. Ecol. Prog. Ser. 349, 289–301. doi: 10.3354/meps07066
Gol’din P., Startsev D. (2017). A systematic review of cetothere baleen whales (Cetacea, Cetotheriidae) from the Late Miocene of Crimea and Caucasus, with a new genus. Papers Palaeontology 3, 49–68. doi: 10.1002/spp2.1066
Goodrich J. S., Sanderson S. L., Batjakas I. E., Kaufman L. S. (2000). Branchial arches of suspension-feeding Oreochromis esculentus: sieve or sticky filter? J. Fish Biol. 56, 858–875. doi: 10.1111/j.1095-8649.2000.tb00877.x
Greenberg D. A., Fudge D. S. (2012). Regulation of hard alpha-keratin mechanics via control of intermediate filament hydration: matrix squeeze revisited. Proc. R. Soc. B 280, e2158. doi: 10.1098/rspb.2012.2158
Hamann L., Blanke A. (2022). Suspension feeders: diversity, principles of particle separation and biomimetic potential. J. R. Soc. Interface 19, e0741. doi: 10.1098/rsif.2021.0741
Hearle J. W. S. (2000). A critical review of the structural mechanics of wool and hair fibres. Int. J. Biol. Macromolecules 27, 123–138. doi: 10.1016/S0141-8130(00)00116-1
Hocking D. P., Marx F. G., Fitzgerald E. M. G., Evans A. R. (2017a). Ancient whales did not filter feed with their teeth. Biol. Lett. 13, 20170348. doi: 10.1098/rsbl.2017.0348
Hocking D. P., Marx F. G., Park T., Fitzgerald E. M. G., Evans A. R. (2017b). A behavioural framework for the evolution of feeding in predatory aquatic mammals. Proc. R. Soc. B 284, e2016750. doi: 10.1098/rspb.2016.2750
Horwood J. (2017). Sei whale, in Encyclopedia of Marine Mammals 3e, ed. Eds. Würsig B., Thewissen J. G. M., Kovacs K. (San Diego: Academic Press), 845–847.
Humphries S. (2009). Filter feeders and plankton increase particle encounter rates through flow regime control. Proc. Natl. Acad. Sci. 106, 7882–7887. doi: 10.1073/pnas.0809063106
Hung T.-C., Piedrahita R. H., Cheer A. (2012). Bio-inspired particle separator design based on the food retention mechanism by suspension-feeding fish. Bioinspiration Biomimetics 7, 046003. doi: 10.1088/1748-3182/7/4/046003
Jain A., De S. (2019). Processing of beverages by membranes, in Processing and Sustainability of Beverages. Eds. Grumezescu A. M., Hoban A. M. (Cambridge UK: Woodhead Publishing), pp 517–pp 560.
Jensen M. M., Saladrigas A. H., Goldbogen J. A. (2017). Comparative three-dimensional morphology of baleen: cross-sectional profiles and volume measurements using CT images. Anatomical Rec. 300, 1942–1952. doi: 10.1002/ar.23648
Kahane-Rapport S. R., Goldbogen J. A. (2018). Allometric scaling of morphology and engulfment capacity in rorqual whales. J. Morphology 279, 1256–1268. doi: 10.1002/jmor.20846
Kahane-Rapport S. R., Savoca M. S., Cade D. E., Segre P. S., Bierlich K. C., Calambokidis J., et al. (2020). Lunge filter feeding biomechanics constrain rorqual foraging ecology across scale. J. Exp. Biol. 223, jeb224196. doi: 10.1242/jeb.224196
Kasuya T., Rice D. W. (1970). Notes on baleen plates and on arrangement of parasitic barnacles of gray whale. Sci. Rep. Whales Res. Institute 22, 39–43.
Kawamura A. (1974). Food and feeding ecology in the southern sei whale. Sci. Rep. Whales Res. Institute 26, 25–144.
Kawamura A. (1980). A review of food of balaenopterid whales. Sci. Rep. Whales Res. Institute 32, 155–198.
Kemper C. M. (2017). “Pygmy right whale,” in Encyclopedia of Marine Mammals 3e, ed. Eds. Würsig B., Thewissen J. G. M., Kovacs K. (Academic Press, San Diego), 790–792.
Kimura T., Ozawa T. (2002). A new cetothere (Ceatacea: Mysticeti) from the early Miocene of Japan. J. Vertebrate Paleontology 22, 684–702. doi: 10.1671/0272-4634(2002)022[0684:ANCCMF]2.0.CO;2
Kitchener A., Vincent J. F. V. (1987). Composite theory and the effect of water on the stiffness of horn keratin. J. Material Sci. 22, 1385–1389. doi: 10.1007/BF01233138
LaBarbera M. (1984). Feeding currents and particle capture mechanisms in suspension feeding animals. Am. Zoologist 24, 71–84. doi: 10.1093/icb/24.1.71
Lambert O., Martínez-Cáceres M., Bianucci G., DiCelma C., Salas-Gismondi R., Steurbaut E., et al. (2017). Earliest mysticete from the Late Eocene of Peru sheds new light on the origin of baleen whales. Curr. Biol. 27, 1535–1541. doi: 10.1016/j.cub.2017.04.026
Lambertsen R. H. (1983). Internal mechanism of rorqual feeding. J. Mammalogy 64, 76–88. doi: 10.2307/1380752
Lambertsen R. H., Hintz R. J., Lancaster W. C., Hirons A., Kreiton K. J., Moor C. (1989). Characterization of the functional morphology of the mouth of the bowhead whale, Balaena mysticetus, with special emphasis on the feeding and filtration mechanisms. Report to the Department of Wildlife Management (Barrow, Alaska: North Slope Borough).
Lambertsen R. H., Rasmussen K. J., Lancaster W. C., Hintz R. J. (2005). Functional morphology of the mouth of the bowhead whale and its implications for conservation. J. Mammalogy 86, 342–352. doi: 10.1644/BER-123.1
Langeland A., Nost T. (1995). Gill raker structure and selective predation on zooplankton by particulate feeding fish. J. Fish Biol. 47, 719–732. doi: 10.1111/j.1095-8649.1995.tb01937.x
Loch C., Viegas S. V., Waddell J. N., Kemper C., Cook R. B., Werth A. J. (2020). Structure and properties of baleen in the Southern Right (Eubalaena australis) and Pygmy Right whales (Caperea marginata). J. Mechanical Behav. Biomed. Materials 110, e103939. doi: 10.1016/j.jmbbm.2020.103939
Lu W. M., Hwang K. J., Ju S. C. (1993). Studies on the mechanism of cross-flow filtration. Chem. Eng. Sci. 48, 863–872. doi: 10.1016/0009-2509(93)80325-K
Lu W. M., Ju S. C. (1989). Selective particle deposition in crossflow filtration. Separation Sci. Technol. 24, 517–540. doi: 10.1080/01496398908049789
Lubetkin S. C., Zeh J. E., Rosa C., George J. C. (2008). Age estimation for young bowhead whales (Balaena mysticetus) using annual baleen growth increments. Can. J. Zoology 86, 525–538. doi: 10.1139/Z08-028
Lysiak N. S. J., Trumble S. J., Knowlton A. R., Moore M. J. (2018). Fishing gear entanglement on a North Atlantic right whale (Eubalaena glacialis) using stable isotopes, steroid and thyroid hormones in baleen. Front. Mar. Sci. 5, e168. doi: 10.3389/fmars.2018.00168
Makabe R., Akamatsu K., Tatsumi R., Koike O., Nakao S. (2021). Numerical simulations of lift force and drag force on a particle in cross-flow microfiltration of colloidal suspensions to understand limiting flux. J. Membrane Sci. 621, 118998. doi: 10.1016/j.memsci.2020.118998
Marshall C. D. (2008). Feeding morphology, in Encyclopedia of Marine Mammals 2e. Eds. Würsig B., Thewissen J. G. M., Kovacs K. (New York: Elsevier), pp 406–pp 414.
Marshall C. D., Goldbogen J. A. (2015). Marine mammal feeding mechanisms, in Marine mammal physiology: requisites for ocean living. Eds. Castellini M. A., Mellish J. A. (Boca Raton FL: CRC), pp 95–pp117.
Marshall R. C., Orwin D. F. G., Gillespie J. M. (1991). Structure and biochemistry of mammalian hard keratin. Electron Microscopy Rev. 4, 47–83. doi: 10.1016/0892-0354(91)90016-6
Marshall C. D., Pyenson N. D. (2019). Feeding in aquatic mammals: an evolutionary and functional approach, in Feeding in vertebrates: anatomy, biomechanics, evolution. Eds. Bels V., Whishaw I. Q. (New York: Springer), pp 743–pp 785.
Marx F. G., Collareta A., Gioncada A., Post K., Lambert O., Bonaccorsi E., et al. (2017). How whales used to filter: exceptionally preserved baleen in a Miocene cetotheriid. J. Anat. 231, 212–220. doi: 10.1111/joa.12622
Marx F. G., Fordyce R. E. (2015). Baleen boom and bust: a synthesis of mysticete phylogeny, diversity and disparity. R. Soc. Open Sci. 2, 1–14. doi: 10.1098/rsos.140434
Marx F. G., Fordyce R. E. (2016). A link no longer missing: new evidence for the cetotheriid affinities of Caperea. PLoS One 11, 0164059. doi: 10.1371/journal.pone.0164059
Marx F. G., Hocking D. P., Park T., Ziegler T., Evans A. R., Fitzgerald E. M. G. (2016a). Suction feeding preceded filtering in baleen whale evolution. Memoirs Museum Victoria 75, 71–82. doi: 10.24199/j.mmv.2016.75.04
Marx F., Lambert O., Uhen M. D. (2016b). Cetacean Paleobiology (London: Wiley-Blackwell). doi: 10.1002/9781118561546
Matyka M., Khalili A., Kozal Z. (2008). Tortuosity–porosity relation in the porous media flow. Phys. Rev. E 78, e026306. doi: 10.1103/PhysRevE.78.026306
Mayo C. A., Letcher B. H., Scott S. (2001). Zooplankton filtering efficiency of the baleen of a North Atlantic right whale, Eubalaena glacialis. J. Cetacean Resource Manage. 2, 225–229. doi: 10.47536/jcrm.vi.286
Mayo C. A., Marx M. K. (1990). Surface foraging behaviour of the North Atlantic right whale, Eubalaena glacialis, and associated zooplankton characteristics. Can. J. Zoology 68, 2214–2220. doi: 10.1139/z90-308
Mchedlidze G. A. (1984). General Features of the Paleobiological Evolution of Cetacea (New Delhi: Oxonian).
McKittrick J., Chen P. Y., Bodde S. G., Yan W., Novitskaya E. E., Meyers M. A. (2012). The structure, functions, and mechanical properties of keratin. J. Mineral Metals Material Soc. 64, 449–468. doi: 10.1007/s11837-012-0302-8
McMillan C. J., Towers J. R., Hildering J. (2019). The innovation and diffusion of “trap-feeding,” a novel humpback whale foraging strategy. Mar. Mammal Sci. 35, 779–796. doi: 10.1111/mms.12557
Mei R. (1992). An approximate expression for the shear lift on a spherical particle at finite Reynolds number. Int. J. Multiphase Flow 18, 145–147. doi: 10.1016/0301-9322(92)90012-6
Michaud J., Taggart C. T. (2007). Lipid and gross energy content of North Atlantic right whale food, Calanus finmarchicus, in the Bay of Fundy. Endangered Species Res. 2007, 77–94. doi: 10.3354/esr003077
Mironov V., Boland T., Trusk G., Forgacs G., Markwald R. R. (2003). Organ printing: computer-aided jet-based 3D tissue engineering. Trends Biotechnol. 21, 157–161. doi: 10.1016/S0167-7799(03)00033-7
Moffat R., Spriggs J., O’Connor S. (2008). The use of baleen for arms, armour, and heraldic crests in medieval Britain. Antiquities J. 88, 207–215. doi: 10.1017/S0003581500001402
Motta P. J., Maslanka M., Hueter R. E., Davis R. L., de la Parra R., Mulvany S. L., et al. (2010). Feeding anatomy, filter-feeding rate, and diet of whale sharks Rhincodon typus during surface ram filter feeding off the Yucatan Peninsula, Mexico. Zoology 113, 199–212. doi: 10.1016/j.zool.2009.12.001
Nemoto T. (1959). Food of baleen whales with reference to whale movements. Sci. Rep. Whales Res. Institute 14, 149–241.
Nemoto T. (1970). Feeding pattern of baleen whales in the ocean, in Marine Food Chains. Ed. Steele J. H. (Edinburgh: Oliver & Boyd), pp 241–pp 252.
Nerini M. (1984). A review of gray whale feeding ecology, in The Gray Whale Eschrichtius robustus. Eds. Jones M. L., Swartz S. L., Leatherwood S. (San Diego: Academic), pp 423–pp 450.
Nousek-McGregor A. E. (2010). “The cost of locomotion in North Atlantic right whales Eubabalena glacialis,” (Durham NC USA: Duke University).
Nowacek D. P., Johnson M., Tyack P., Shorter K. A., McLellan W. A., Pabst D. A. (2001). Buoyant balaenids: the ups and downs of buoyancy in right whales. Proc. R. Soc. London 268, 1811–1816. doi: 10.1098/rspb.2001.1730
Orton L. S., Brodie P. F. (1987). Engulfing mechanisms of fin whales. Can. J. Zoology 65, 2898–2907. doi: 10.1139/z87-440
Paig-Tran E. W. M., Bizzarro J. J., Strother J. A., Summers A. P. (2011). Bottles as models: predicting the effects of varying swimming speed and morphology on size selectivity and filtering efficiency in fishes. J. Exp. Biol. 214, 1643–1654. doi: 10.1242/jeb.048702
Paig-Tran E. W. M., Kleinteich T., Summers A. P. (2013). The filter pads and filtration mechanisms of the devil rays: variation at macro and microscopic scales. J. Morphology 274, 1026–1043. doi: 10.1002/jmor.20160
Paig-Tran E. W. M., Summers A. P. (2014). Comparison of the structure and composition of the branchial filters in suspension feeding elasmobranchs. Anatomical Rec. 297, 701–715. doi: 10.1002/ar.22850
Pautard F. G. E. (1963). Mineralization of keratin and its comparison with the enamel matrix. Nature 199, 531–535. doi: 10.1038/199531a0
Peng J., Dabiri J. O. (2009). Transport of inertial particles by Lagrangian coherent structures: Application to predator–prey interaction in jellyfish feeding. J. Fluid Mechanics 623, 75–84. doi: 10.1017/S0022112008005089
Peredo C. M., Pyenson N. D., Boersma A. T. (2017). Decoupling tooth loss from the evolution of baleen in whales. Front. Mar. Sci. 4, 1–11. doi: 10.3389/fmars.2017.00067
Pfeiffer C. (1992). Cellular structure of terminal baleen in various mysticete species. Aquat. Mammals 18, 67–73.
Pinto S. J. D., Shadwick R. E. (2013). Material and structural properties of fin whale (Balaenoptera physalus) zwischensubstanz. J. Morphology 274, 947–955. doi: 10.1002/jmor.20154
Pivorunas A. (1976). A mathematical consideration of the function of baleen plates and their fringes. Sci. Rep. Whales Res. Institute 28, 37–55.
Pivorunas A. (1979). The feeding mechanisms of baleen whales. Am. Scientist 67, 432–440. Available at: https://www.jstor.org/stable/27849332.
Pomerleau C., Matthews C. J. D., Gobeil C., Stern G. A., Ferguson S. H., Macdonald R. W. (2018). Mercury and stable isotope cycles in baleen plates are consistent with year-round feeding in two bowhead whale (Balaena mysticetus) populations. Polar Biol. 41, 1–13. doi: 10.1007/s00300-018-2329-y
Potvin J., Cade D. E., Werth A. J., Shadwick R. E., Goldbogen J. A. (2020). A perfectly inelastic collision: bulk prey engulfment by baleen whales and dynamical implications for the world’s largest cetaceans. Am. J. Phys. 2020, e1771. doi: 10.1119/10.0001771
Potvin J., Cade D. E., Werth A. J., Shadwick R. E., Goldbogen J. A. (2021). Rorqual lunge-feeding energetics near and away from the kinematic threshold of optimal efficiency. Integr. Comp. Biol. 3, obab005. doi: 10.1093/iob/obab005
Potvin J., Goldbogen J. A., Shadwick R. E. (2009). Passive versus active engulfment: verdict from trajectory simulations of lunge-feeding fin whales Balaenoptera physalus. J. R. Soc. Interface 6, 1005–1025. doi: 10.1098/rsif.2008.0492
Potvin J., Goldbogen J. A., Shadwick R. E. (2012). Metabolic expenditures of lunge feeding rorquals across scale: implications for the evolution of filter feeding and the limits to maximum body size. PLoS One 7, 44854. doi: 10.1371/journal.pone.0044854
Potvin J., Werth A. J. (2017). Oral cavity hydrodynamics and drag production in balaenid whale suspension feeding. PLoS One 12, 5220. doi: 10.1371/journal.pone.0175220
Potvin J., Werth A. J. (2024). Suffused: baleen fringe mat porosity and hydrodynamics in balaenid and balaenopterid whales. Biol. J. Linn. Soc. in press. doi: 10.1093/biolinnean/blae030
Pyenson N. D. (2017). The ecological rise of whales chronicled by the fossil record. Curr. Biol. 27, 558–564. doi: 10.1016/j.cub.2017.05.001
Pyenson N. D. (2018). Spying on Whales: the Past, Present, and Future of Earth’s Most Awesome Creatures (New York: Penguin Random House).
Pyenson N. D., Vermeij G. J. (2016). The rise of ocean giants: maximum body size in Cenozoic marine mammals as an indicator for productivity in the Pacific and Atlantic Oceans. Biol. Lett. 12, 20160186. doi: 10.1098/rsbl.2016.0186
Ray G. C., Schevill W. E. (1974). Feeding of a captive gray whale, Eschrichtius robustus. Mar. Fisheries Rev. 36, 31–38.
Rice D. W., Wolman A. A. (1971). The life history and ecology of the gray whale (Eschrichtius robustus). Am. Soc. Mammalogy 3, 1–142. doi: 10.5962/bhl.title.39537
Ridgway S. H., Harrison R. (1999). Handbook of Marine Mammals, Volume 6: Second Book of dolphins and porpoises (San Diego: Academic).
Ripperger S., Altmann J. (2002). Crossflow microfiltration—state of the art. Separation Purification Technol. 26, 19–31. doi: 10.1016/S1383-5866(01)00113-7
Rubenstein D. I., Koehl M. A. R. (1977). The mechanisms of filter feeding: some theoretical considerations. Am. Nat. 111, 981–994. doi: 10.1086/283227
Ruud J. T. (1940). The surface structure of the baleen plates and a possible clue to age in whales. Hvalradets Skrifter 23, 1–24.
Sanderson S. L., Cheer A. Y., Goodrich J. S., Graziano J. D., Callan W. T. (2001). Crossflow filtration in suspension-feeding fishes. Nature 412, 439–441. doi: 10.1038/35086574
Sanderson S. L., Roberts E., Lineburg J., Brooks H. (2016). Fish mouths as engineering structures for vortical cross-step filtration. Nat. Commun. 7, e11092. doi: 10.1038/ncomms11092
Sanderson S. L., Wassersug R. L. (1990). Suspension feeding vertebrates. Sci. Am. 262, 96–101. doi: 10.1038/scientificamerican0390-96
Sanderson S. L., Wassersug R. (1993). Convergent and alternative designs for vertebrate suspension feeding, in The Skull Volume 3: Functional and Evolutionary Mechanisms. Eds. Hanken J., Hall B. K. (Chicago: University of Chicago Press), pp 37–pp112.
Savoca M. S., Czapanskiy M. F., Kahane-Rapport S. R., Gough W. T., Fahlbusch J. A., Bierlich K. C., et al. (2021). Baleen whale prey consumption based on high-resolution foraging measurements. Nature 599, 85–90. doi: 10.1038/s41586-021-03991-5
Schroeder A., Marshall L., Trease B., Becker A., Sanderson S. L. (2019). Development of helical, fish-inspired cross-step filter for collecting harmful algae. Bioinspiration Biomimetics 14, 056008. doi: 10.1088/1748-3190/ab2d13
Sears R., Perrin W. F. (2008). “Blue whales,” in Encyclopedia of marine mammals 2e. Eds. Würsig B., Thewissen J. G. M., Kovacs K. (Elsevier, New York), pp 120–pp 124.
Segre P. S., Weir C. R., Stanworth A., Cartwright S., Friedlaender A. S., Goldbogen J. A. (2021). Biomechanically distinct filter-feeding behaviors distinguish sei whales as a functional intermediate and ecologically flexible species. J. Exp. Biol. 224, e238873. doi: 10.1242/jeb.238873
Sekiguchi K., Best P. B., Kaczmaruk B. Z. (1992). New information on the feeding habits and baleen morphology of the pygmy right whale Caperea marginata. Mar. Mammal Sci. 8, 288–293. doi: 10.1111/j.1748-7692.1992.tb00411.x
Shadwick R. E., Goldbogen J. A., Potvin J., Pyenson N. D., Vogl A. W. (2013). Novel muscle and connective tissue design enables high extensibility and controls engulfment volume in lunge-feeding rorqual whales. J. Exp. Biol. 216, 2691–2701. doi: 10.1242/jeb.081752
Shadwick R. E., Potvin J., Goldbogen J. A. (2019). Lunge feeding in rorqual whales. Physiology 34, 409–418. doi: 10.1152/physiol.00010.2019
Shimeta J., Jumars P. A. (1991). Physical mechanisms and rates of particle capture by suspension-feeders. Oceanography Mar. Biol. Annu. Rev. 29, 191–257.
Sibanda V., Greenwood R. W., Seville J. P. K. (2001). Particle separation from gases using cross-flow filtration. Powder Technol. 118, 193–202. doi: 10.1016/S0032-5910(01)00311-4
Simon M., Johnson M., Madsen P. T. (2012). Keeping momentum with a mouthful of water: behavior and kinematics of humpback whale lunge feeding. J. Exp. Biol. 215, 3786–3798. doi: 10.1242/jeb.071092
Simon M., Johnson M., Tyack P., Madsen P. T. (2009). Behaviour and kinematics of ram filtration in bowhead whales (Balaena mysticetus). Proc. R. Soc. B 276, 3819–3828. doi: 10.1098/rspb.2009.1135
Sims D. W. (2008). Sieving a living: a review of the biology, ecology and conservation status of the plankton-feeding basking shark Cetorhinus maximus. Adv. Mar. Biol. 54, 171–220. doi: 10.1016/S0065-2881(08)00003-5
Slater G. J., Goldbogen J. A., Pyenson N. D. (2017). Independent evolution of baleen whale gigantism linked to Plio-Pleistocene ocean dynamics. Proc. R. Soc. B 284, 20170546. doi: 10.1098/rspb.2017.0546
Smith J. C., Sanderson S. L. (2008). Intra-oral flow patterns and speeds in a suspension-feeding fish with gill rakers removed versus intact. Biol. Bull. 215, 309–318. doi: 10.2307/25470714
Song L., Elimelech M. (1995). Theory of concentration polarization in cross-flow filtration. J. Chem. Soc. Faraday Trans. 91, 3389–3398. doi: 10.1039/ft9959103389
St. Aubin D. J., Stinson R. H., Geraci J. R. (1984). Aspects of the structure and composition of baleen, and some effects of exposure to petroleum hydrocarbons. Can. J. Zoology 62, 193–198. doi: 10.1139/z84-032
Stevenson C. H. (1907). Whalebone: its production and utilization. Bureau Fisheries Documents 626, 1–12. Available at: http://hdl.loc.gov/loc.gdc/scd0001.00028900855.
Sumich J. L. (2001). Growth of baleen in a rehabilitating gray whale calf. Aquat. Mammals 27, 234–238.
Sutherland K. (2005). The A-Z of filtration. Filtration separation 42, 40. doi: 10.1016/S0015-1882(05)70731-X
Szewciw L. J., de Kerkhove D. G., Grime G. W., Fudge D. S. (2010). Calcification provides mechanical reinforcement to whale baleen alpha keratin. Proc. R. Soc. B. 277 (1694), 2597–2605. doi: 10.1098/rspb.2010.0399
Tamime A. Y. (2013). “Membrane PRocessing: Dairy and Beverage Applications,” (Hoboken NJ: Wiley/Blackwell).
Taylor A. M., Bonser R. H. C., Farrent J. W. (2004). The influence of hydration on the tensile and compressive properties of avian keratinous tissues. J. Materials Sci. 39, 939–942. doi: 10.1023/B:JMSC.0000012925.92504.08
Thewissen J. G. M. (1998). The emergence of Whales: Evolutionary Patterns in the Origins of Cetacea (New York: Plenum). doi: 10.1007/978-1-4899-0159-0
Thewissen J. G. M., Bajpai S. (2001). Whale origins as a poster child for macroevolution. BioScience 51, 1037–1049. doi: 10.1641/0006-3568(2001)051[1037:WOAAPC]2.0.CO;2
Thewissen J. G. M., Cooper L. N., George J. C., Bajpai S. (2009). From land to water: the origin of whales, dolphins, and porpoises. Evolution: Educ. Outreach 2, 272–288. doi: 10.1007/s12052-009-0135-2
Thewissen J. G. M., Hieronymus T. L., George J. C., Suydam R., Stimmelmayr R., McBurney D. (2017). Evolutionary aspects of the development of teeth and baleen in the bowhead whale. J. Anat. 230, 549–566. doi: 10.1111/joa.12579
Trakumas S., Willeke K., Reponen T., Grinshpun S. A., Friedman W. (2001). Comparison of filter bag, cyclonic, and wet dust collection methods in vacuum cleaners. Am. Ind. Hygiene Assoc. J. 62, 573–583. doi: 10.1080/15298660108984656
Tsai C.-H., Fordyce R. E. (2015). The earliest gulp-feeding mysticete from the Oligocene of New Zealand. J. Mamm. Evol. 22, 535–560. doi: 10.1007/s10914-015-9290-0
Uhen M. D. (2010). The origin(s) of whales. Annu. Rev. Earth Planetary Sci. 38, 189–219. doi: 10.1146/annurev-earth-040809-152453
Vandenberg M. L., Cohen K. E., Rubin R. D., Goldbogen J. A., Summer A. P., Paig-Tran E. W. M., et al. (2023). Formation of a fringe: a look inside baleen morphology using a multimodal visual approach. J. Morphology 284, e21574. doi: 10.1002/jmor.21574
van der Hoop J. M., Corkeron P., Kenney J., Landry S., Morin D., Smith J., et al. (2015). Drag from fishing gear entangling North Atlantic right whales. Mar. Mammal Sci. 32, 619–642. doi: 10.1111/mms.12292
van der Hoop J. M., Nousek-McGregor A. E., Nowacek D. P., Parks S. E., Tyack P., Madsen P. T. (2019). Foraging rates of ram-filtering North Atlantic right whales. Funct. Ecol. 33, 1290–1306. doi: 10.1111/1365-2435.13357
Vogel S. (2013). Comparative biomechanics: life’s physical world 2e. (Princeton: Princeton University Press).
Vogel H. C., Todaro C. M. (1997). Cross flow filtration, in fermentation and biochemical engineering handbook, ed. Bhave RR, pp 271–322, New York: Elsevier (Noyes).
Wang B., Yan W., McKittrick J., Meyers M. A. (2016). Keratin: structure, mechanical properties, occurrence in biological organisms, and efforts at bioinspiration. Prog. Materials Sci. 76, 229–318. doi: 10.1016/j.pmatsci.2015.06.001
Watkins W. A., Schevill W. E. (1976). Right whale feeding and baleen rattle. J. Mammalogy 57, 58–66. doi: 10.2307/1379512
Webber M. A., Keener W., Markowitz T. M., Chamberlin D., Allen D., Lane R. S., et al. (2024). Fish feeding and rapid foraging behavior switching by gray whales (Eschrichtius robustus) in California. Aquat. Mammals 50, 132–151. doi: 10.1578/AM.50.2.2024.132
Wegner N. C. (2015). Elasmobranch gill structure Vol. 34 (San Diego, CA, USA: Fish Physiology), 101–151.
Werth A. J. (2000). Marine mammals, in Feeding: form, function and evolution in tetrapod vertebrates. Ed. Schwenk K. (New York: Academic), pp 475–pp 514.
Werth A. J. (2001). How do mysticetes remove prey trapped in baleen? Bull. Museum Comp. Zoology 156, 189–203.
Werth A. J. (2004). Models of hydrodynamic flow in the bowhead whale filter feeding apparatus. J. Exp. Biol. 207, 3569–3580. doi: 10.1242/jeb.01202
Werth A. J. (2007). Adaptations of the cetacean hyolingual apparatus for aquatic feeding and thermoregulation. Anatomical Rec. 290, 546–568. doi: 10.1002/ar.20538
Werth A. J. (2012). Hydrodynamic and sensory factors governing response of copepods to simulated predation by baleen whales. Int. J. Ecol. 2012, 208913. doi: 10.1155/2012/208913
Werth A. J. (2013). Flow-dependent porosity of baleen from the bowhead whale (Balaena mysticetus). J. Exp. Biol. 216, 1152–1159. doi: 10.1242/jeb.078931
Werth A. J. (2017). Baleen, in Encyclopedia of Marine Mammals 3e. Eds. Würsig B., Thewissen J. G. M., Kovacs K. (San Diego: Elsevier), pp 60–pp 61.
Werth A. J. (2019). “Variable porosity of throughput and tangential filtration in biological and 3D printed systems,” in Advances in Engineering Research, vol. 29 . Ed. Petrova V. (Nova Science Publishers, Hauppauge, NY), pp 37–pp 93. Porosity—Properties and Measurement.
Werth A. J., Blakeney S. M., Cothren A. I. (2019a). Oil adsorption does not structurally or functionally alter whale baleen. R. Soc. Open Sci. 6, 182194. doi: 10.1098/rsos.182194
Werth A. J., Crompton A. W. (2023). Cetacean tongue mobility and function: a comparative review. J. Anat. 243, 343–373. doi: 10.1111/joa.13876
Werth A. J., Harriss R. W., Rosario M. V., George J. C., Sformo T. L. (2016a). Hydration affects the physical and mechanical properties of baleen tissue. R. Soc. Open Sci. 3, 160591. doi: 10.1098/rsos.160591
Werth A. J., Ito H. (2017). Sling, scoop, squirter: anatomical features facilitating prey transport, concentration, and swallowing in rorqual whales (Mammalia: Mysticeti). Anatomical Rec. 300, 2070–2086. doi: 10.1002/ar.23606
Werth A. J., Kahane-Rapport S. R., Potvin J., Goldbogen J. A., Savoca M. S. (2024). Baleen–plastic interactions reveal high risk to all filter-feeding whales from clogging, ingestion, and entanglement. Oceans 5, 48–70. doi: 10.3390/oceans5010004
Werth A. J., Kosma M. A., Chenoweth E. M., Straley J. M. (2019b). New views of humpback whale flow dynamics and morphology during prey engulfment. Mar. Mammal Sci. 35, 1556–1578. doi: 10.1111/mms.12614
Werth A. J., Marshall C. D. (2023). “Convergent evolution of secondarily aquatic feeding in mammals,” in Convergent Evolution. Eds. Bels V., Legreneur P. (Springer, Cham, Switzerland), pp 183–pp 220.
Werth A. J., Potvin J. (2016). Baleen hydrodynamics and morphology of cross-flow filtration in balaenid whale suspension feeding. PLoS One 11, e0150106. doi: 10.1371/journal.pone.0150106
Werth A. J., Potvin J., Shadwick R. E., Jensen M. M., Cade D. E., Goldbogen J. A. (2018a). Filtration area scaling and evolution in mysticetes: trophic niche partitioning and the curious cases of the sei and pygmy right whales. Biol. J. Linn. Soc. 125, 264–279. doi: 10.1093/biolinnean/bly121
Werth A. J., Rita Espada D., Rosario M. V., Moore M. J., Sformo T. L. (2018b). How do baleen whales stow their filter: a comparative biological analysis. J. Exp. Biol. 221, 189233. doi: 10.1242/jeb.189233
Werth A. J., Sformo T. L. (2020). “Anatomy and function of feeding,” in The Bowhead Whale, Balaena mysticetus: Biology and Human Interactions. Eds. George J. C., Thewissen J. G. M. (Academic, San Diego), pp 213–pp 223.
Werth A. J., Sformo T. L., Lysiak N. S., Rita Espada D., George J. C. (2020). Baleen turnover and gut transit in mysticete whales and its environmental implications. Polar Biol. 43, 707–723. doi: 10.1007/s00300-020-02673-8
Werth A. J., Sformo T. L., Lysiak N. S., Rita Espada D., George J. C. (2021). Differential baleen growth and its consequences. Polar Biol. 44, 1227–1228. doi: 10.1007/s00300-021-02878-5
Werth A. J., Straley J., Shadwick R. (2016b). Baleen wear reveals intraoral water flow patterns of mysticete filter feeding. J. Morphology 277, 453–471. doi: 10.1002/jmor.20510
Werth A. J., van de Graaf P., DesJardins R. (2019c). Preparation of full baleen racks for long-term exhibition and research. Aquat. Mammals 45, 500–506. doi: 10.1578/AM.45.5.2019.500
Werth A. J., Whaley H. R. (2019). Ocean acidification’s potential effects on keratin protein in cetacean baleen and other integumentary tissue. Ann. Ecol. Environ. Sci. 3, 21–28.
Williamson G. R. (1973). Counting and measuring baleen and ventral grooves of whales. Scientific Reports of the Whales Research Institute 24, 279–292.
Witkop E. M., Van Wassenbergy S., Heideman P. D., Sanderson S. L. (2023). Biomimetic models of fish gill rakers as lateral displacement arrays for particle separation. Bioinspiration Biomimetics 18, 056009. doi: 10.1088/1748-3190/acea0e
Woodward B. L., Winn J. P. (2006). Apparent lateralized behavior in gray whales feeding off the central British Columbia coast. Mar. Mammal Sci. 22, 64–73. doi: 10.1111/j.1748-7692.2006.00006.x
Yeong W.-Y., Chua C.-K., Leong K.-F., Chandrasekaran M. (2004). Rapid prototyping in tissue engineering: challenges and potentials. Trends Biotechnol. 22, 643–652. doi: 10.1016/j.tibtech.2004.10.004
Young S. (2012). The comparative anatomy of baleen: evolutionary and ecological implications. San Diego State University, San Diego.
Young S., Deméré T. E., Ekdale E. G., Berta A., Zellmer N. (2015). Morphometrics and structure of complete baleen racks in gray whales (Eschrichtius robustus) from the Eastern North Pacific Ocean. Anatomical Rec. 298, 703–719. doi: 10.1002/ar.23108
Zeman L. J., Zydney A. L. (1996). Microfiltration and Ultrafiltration: Principles and Applications (New York: Dekker).
Zhong P. S., Chung T.-S., Jeyaseelan K., Armugan A. (2012). Aquaporin-embedded biomimetic membranes for nanofiltration. J. Membrane Sci. 407–408, 27–33. doi: 10.1016/j.memsci.2012.03.033
Zhu Y., Hu D., Guo Y., Ding H., Yang G. (2023). Bio-inspired filter design based on vortex control mechanism of parallel groove structure. J. Bionic Eng. 20, 338–348. doi: 10.1007/s42235-022-00247-4
Zhu Y., Hu D., Li C., Zhuang C., Yang G. (2021). CFD-DEM simulation of the hydrodynamic filtration performance in balaenid whale feeding. Sci. Total Environ. 787, 147696. doi: 10.1016/j.scitotenv.2021.147696
Zhu Y., Hu D., Yang G. (2020a). Theoretical analysis of the hydrodynamic filtering system in the balaenid whales suspension feeding. Bioinspiration Biomimetics 16, e26006. doi: 10.1088/1748-3190/abc493
Keywords: filter, flow, baleen, keratin, mysticete, biomechanics
Citation: Werth AJ and Potvin J (2024) Dynamic filtration in baleen whales: recent discoveries and emerging trends. Front. Mar. Sci. 11:1347497. doi: 10.3389/fmars.2024.1347497
Received: 30 November 2023; Accepted: 26 March 2024;
Published: 12 April 2024.
Edited by:
Marco Ghisalberti, University of Western Australia, AustraliaReviewed by:
Michelangelo Bisconti, University of Turin, ItalyLeandra Hamann, University of Florida, United States
Copyright © 2024 Werth and Potvin. This is an open-access article distributed under the terms of the Creative Commons Attribution License (CC BY). The use, distribution or reproduction in other forums is permitted, provided the original author(s) and the copyright owner(s) are credited and that the original publication in this journal is cited, in accordance with accepted academic practice. No use, distribution or reproduction is permitted which does not comply with these terms.
*Correspondence: Alexander J. Werth, awerth@hsc.edu
†ORCID: Alexander J. Werth, orcid.org/0000-0002-7777-478X
Jean Potvin, orcid.org/0000-0002-8071-8340