- 1Department of Oncology, Fourth People’s Hospital in Shenyang, China Medical University, Shenyang, China
- 2Department of Gastroenterology, The First Affiliated Hospital of Dalian Medical University, Dalian Medical University, Dalian, China
C1GALT1 plays a pivotal role in colorectal cancer (CRC) development and progression through its involvement in various molecular mechanisms. This enzyme is central to the O-glycosylation process, producing tumor-associated carbohydrate antigens (TACA) like Tn and sTn, which are linked to cancer metastasis and poor prognosis. The interaction between C1GALT1 and core 3 synthase is crucial for the synthesis of core 3 O-glycans, essential for gastrointestinal health and mucosal barrier integrity. Aberrations in this pathway can lead to CRC development. Furthermore, C1GALT1's function is significantly influenced by its molecular chaperone, Cosmc, which is necessary for the proper folding of T-synthase. Dysregulation in this complex interaction contributes to abnormal O-glycan regulation, facilitating cancer progression. Moreover, C1GALT1 affects downstream signaling pathways and cellular behaviors, such as the epithelial-mesenchymal transition (EMT), by modifying O-glycans on key receptors like FGFR2, enhancing cancer cell invasiveness and metastatic potential. Additionally, the enzyme's relationship with MUC1, a mucin protein with abnormal glycosylation in CRC, highlights its role in cancer cell immune evasion and metastasis. Given these insights, targeting C1GALT1 presents a promising therapeutic strategy for CRC, necessitating further research to develop targeted inhibitors or activators. Future efforts should also explore C1GALT1's potential as a biomarker for early diagnosis, prognosis, and treatment response monitoring in CRC, alongside investigating combination therapies to improve patient outcomes.
1 Introduction
Colorectal cancer (CRC) ranks as the fourth deadliest cancer worldwide, causing nearly 900,000 deaths annually, which accounts for approximately 10% of all annually diagnosed cancers and cancer-related deaths worldwide (1). The incidence and mortality rates vary geographically, with the highest rates seen in the most developed countries (Figure 1). Disturbingly, projections indicate a 60% increase in the global burden of CRC by 2030, with an estimated 2.2 million new cases and 1.1 million deaths (2). While advancements in endoscopic technology and screening methods, along with increased early detection efforts, have led to a decline in CRC incidence (3). However, epidemiological studies highlight a worrying trend of increasing colorectal cancer incidence among individuals under 50 years of age (4, 5). Thus, the significant threat posed by colorectal cancer to human health cannot be underestimated. Numerous risk factors contribute to the development of colorectal cancer, including age, male gender, family history, inflammatory bowel disease, smoking, excessive alcohol consumption, high intake of red and processed meat, obesity, and diabetes (6–8). These risk factors often co-occur and interact with each other. Treatment approaches typically involve early surgical intervention for improved prognosis, while stage II and III rectal cancer patients often require surgery-assisted chemotherapy or radiotherapy (7, 9). Notably, surgery combined with targeted chemotherapy has proven effective in treating advanced metastatic disease or post-surgical recurrence (9). Consequently, identifying reliable targets for colorectal cancer represents a promising avenue to enhance survival rates.
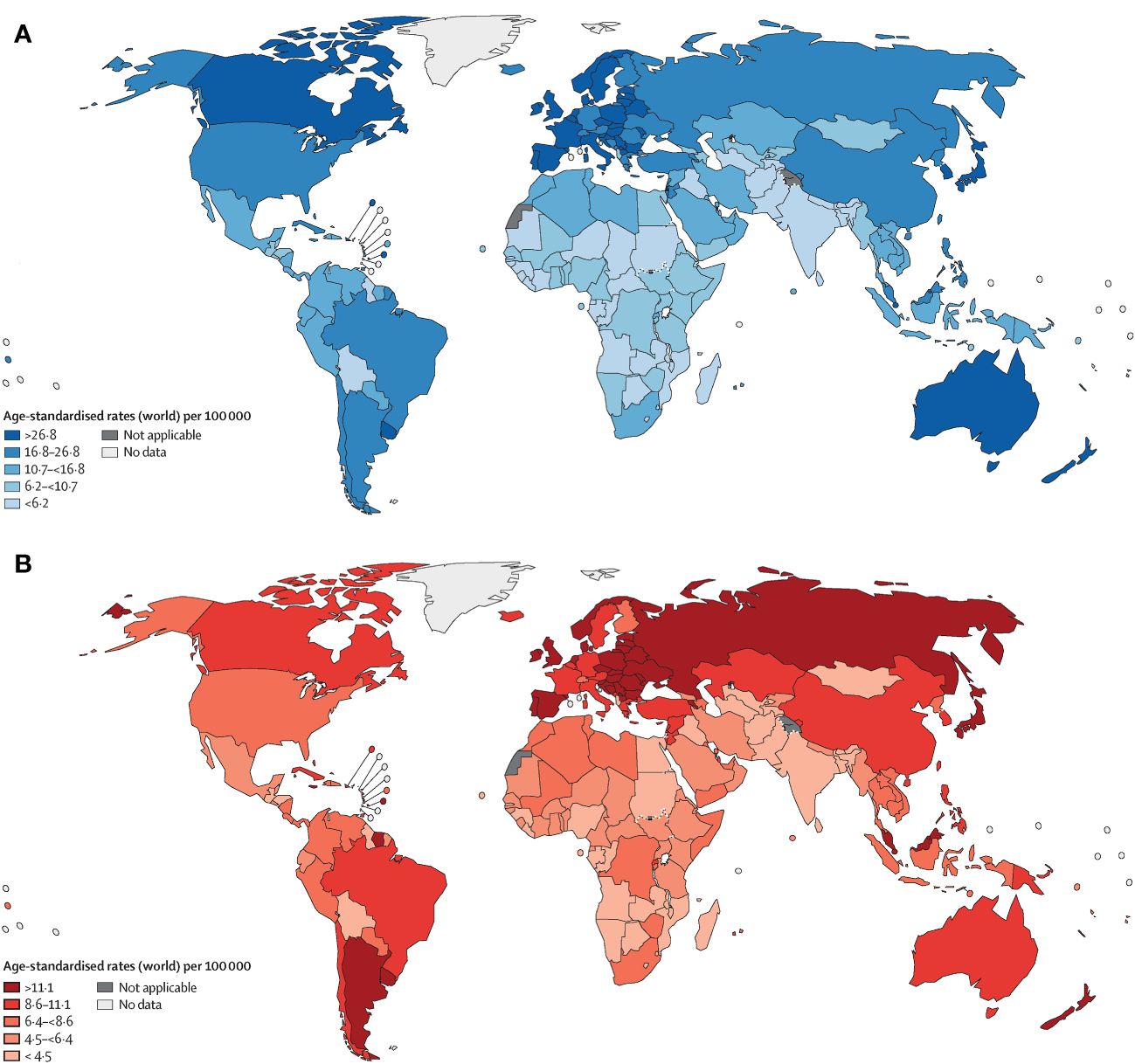
Figure 1 Age-standardised rates of cancer incidence (A) and mortality (B) across countries in five continents, based on the most recent figures from the WHO International Agency for Research on Cancer (1).
Genetic and epigenetic changes are widely recognized as the primary drivers of cancer development, with downstream phenotypic alterations at the protein level playing a crucial role in cancer progression and transmission (10). The alterations includes increased branching of complex and hybrid N-glycans, increased levels of sialyl lewis antigens, truncated O-glycan expression, and complex core fucosylation, which causes anormal expressions of membrane-localized glycans and then leads to malignant transformation in cells (11). Glycosylation, a post-translational modification of proteins, involves the transfer of sugar molecules to proteins through the action of glycosyltransferases, forming glycosidic bonds with amino acid residues (12). It is the most abundant and diverse form of post-translational modification found in all eukaryotic cells (13). Glycopeptide bonds can be categorized into N-linked, O-linked, and C-linked glycosylation, as well as C-mannosylation and generation of GPI-anchored proteins, based on the properties of the linked glycopeptide bonds and oligosaccharides (13). Carbohydrates in the form of N-linked or O-linked oligosaccharides are major structural components of membrane-bound and secreted proteins (14, 15). Through the regulation of protein stability, subcellular localization, activity, and interactions, glycosylation plays a wide-ranging role in key cellular processes, including gene transcription, cell cycle regulation, DNA repair, apoptosis, virus budding, receptor endocytosis, and various physiological and pathological processes (16–18). Tumor cells exhibit extensive glycosylation changes compared to untransformed cells, and glycosylation has been closely associated with various cancers, including hepatocellular cancer (19–21), pancreatic cancer (22, 23), gastric cancer (24), bladder cancer (25), breast cancer (21), esophageal cancer (26), cholangiocarcinoma (27) etc. In colorectal cancer (CRC), glycosylation can impact cell migration, intercellular adhesion, actin polymerization, mitosis, cell membrane repair, apoptosis, cell differentiation, stem cell regulation, intestinal mucosal barrier integrity, immune system regulation, T cell polarization, and intestinal microbiota composition (28, 29). These functions are closely linked to the prognosis and development of CRC, including tumor occurrence, metastasis, immune regulation, and resistance to anti-tumor treatments (28–32). C1GALT1 (T-synthase), a key enzyme in the glycosylation process, has been identified as playing a role in colorectal carcinogenesis in recent years (33, 34). Given the well-established significance of glycosylation in cancer, this article aims to review the role of C1GALT1 in the complex glycosylation process and its relationship with colorectal cancer, providing evidence for the identification of specific therapeutic targets for CRC.
2 C1GALT1 in glycosylation
C1GALT1 (T-synthase) is a crucial mucin-type O-glycosyltransferase that functions as a type II transmembrane glycoprotein with endoplasmic reticulum (ER) and Golgi lumen-oriented catalytic domains (13, 35). As a glycosyltransferase, it plays a key role in the formation of the core 1 structure (Galβ1-3GalNAcα1-O-Ser/Thr) (33, 34). In most normal cells, C1GALT1 is essential for the immediate elongation and processing of GalNAc-type protein O-glycosylation (36). O-GalNAc glycans, also known as mucin O-glycans, are one of the most common post-translational modifications (18, 37). The sugars present in O-GalNAc glycans include GalNAc, Gal, GlcNAc, Fuc, and Sia, while Man, Glc, or Xyl residues are not expressed (37). The initial and fundamental step of O-GalNAc glycosylation involves the addition of α-linked GalNAc to Ser or Thr residues through a series of enzymes known as GalNAcTs3 or ppGalNAts or GALNT, resulting in the production of the Tn antigen within the Golgi apparatus. Subsequently, C1GALT1 catalyzes the formation of the core 1 structure (14, 33, 34, 37, 38). C1GALT1 is responsible for adding β 1-3 glycosidic bonds between Gal and GalNAc Ser/Thr residues to generate the T antigen (the core 1 structure) (25). The T antigen serves as a precursor for the subsequent extension and maturation of mucin-type O-glycans (24, 39). The Tn antigen consists of a single GalNAc residue connected to Ser/Thr α-O-Ser/Thr, serves as the foundation for the extension of O-glycans into various complex branching structures through consecutive glycosyltransferase reactions (Figure 2). The Tn antigen may continue to be catalyzed by ST6GalNAc, which will generate sialyl Tn antigen (sTn) (40, 41).However, the Tn antigen without sialic acid modification will be modified by C1GALT1 (core 1 synthase).The C1GALT1 modification process is to add a Gal to Tn antigen in order to form the T antigen (42). In normal tissues, Tn or T antigens continue to synthesize complex O-glycans with the catalysis of glycoenzymes. Consequently, the Tn antigen is uncommon in normal mucins but is often found at increased levels in tumor mucins (43), and its presence has been closely associated with poor prognosis and low overall survival rates (39).
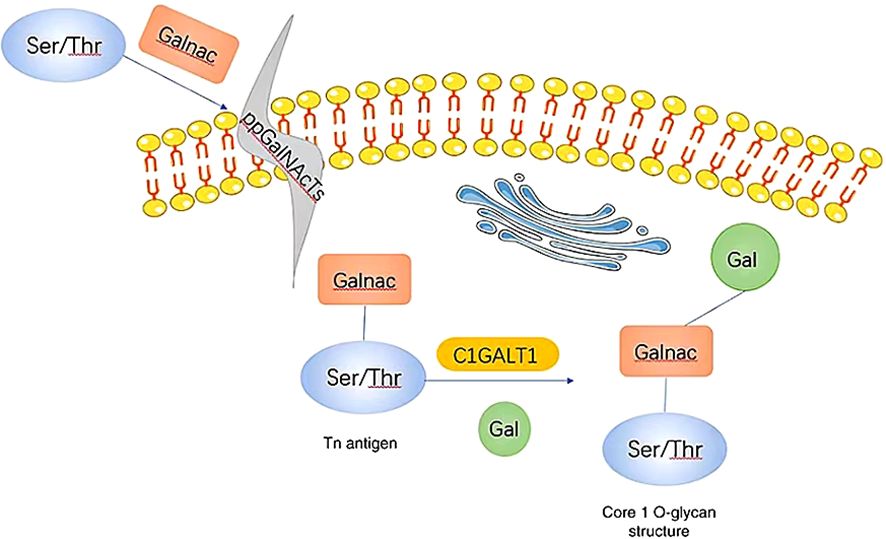
Figure 2 When GalNAc transferase is present, it facilitates the formation of a GalNAc α1-Ser/Thr structure (also referred to as the Tn antigen) with GalNAc on serine/threonine residues. Subsequently, C1GALT1, a core 1β1,3-galactosyltransferase, catalyzes the addition of Gal from UDP-Gal to the Tn antigen, resulting in the creation of a core 1 O-glycan structure. Gal, galactose; GalNAc, N-acetylgalactosamine; Tn, Thomsen-nouvelle; C1GALT1, core 1 β1,3-galactosyltransferase (33).
C1GALT1 enzyme activity requires the presence and function of the molecular chaperone COSMC, which is located in the ER (44). In the ER, C1GALT1 is converted into its active and dimeric forms by the molecular chaperone COSMC before entering the Golgi matrix (45). Within the Golgi apparatus, C1GALT1 competes with two other types of glycosyltransferases (C3GnT and ST6GalNAC-I/II) to catalyze the addition of Gal to GalNAc α-Ser/Thr, initiating O-linked Mucin Glycan Formation and the Core-1 Structure (46).
The Tn antigen (GalNAcα 1-O-Ser/Thr) is an O-glycan commonly expressed in various types of human cancers, often resulting from incomplete glycosylation (47). It belongs to the category of tumor-associated carbohydrate antigens (TACA) found in human cancer. The most common TACAs formed from incomplete synthesis are GalNAcα-O-Ser/Thr (Tn, Thomsen Nouveau, CD175), Galβ1,3-GalNAcα-O-Ser/Thr (TF, Thomsen-Friedenreich, CD176, T antigen), Neu5Acα2,6-GalNAcα-O-Ser/Thr (sTn, sialyl Tn, CD175s) and Neu5Acα2,6- and Neu5Acα2,3-Galβ1,3-GalNAcα-O-Ser/Thr (2,6-sTF, 2,3-sTF) (47). In normal tissues, the Tn antigen is typically undetectable due to its efficient conversion into a broader range of glycans, primarily the core 1 structure (Galβ1-3GalNAcα-O-Ser/Thr, T or TF antigen) (43). The core 1 structure can further extend into the extended core 1 O-glycan (core 1 O-glycan), or branch into the core 2 structure or undergo sialylation (48). In the gastrointestinal tract (GI tract), the Tn antigen can be converted into the core 3 structure (34, 49), while in the normal colon, the primary O-glycan core structure is the core 3 structure (50). The core 4 structure is formed by adding Uridine Diphosphate (UDP) from the core 3 precursor to the core 3 infrastructure through C2GnT2-α-D-GlcNAcβ1,6-GlcNAc (51). As described above, Mucin-type O-glycan core structures and key sialoglycoforms such as the Tn antigen, the core 1 structure are shown in Figure 3 and the process of structure formation is shown in Figure 4. However, incomplete glycosylation caused by various factors can lead to the production of TACAs as described above. These truncated O-glycans possess carcinogenic characteristics and can directly induce cell growth and invasion (52).
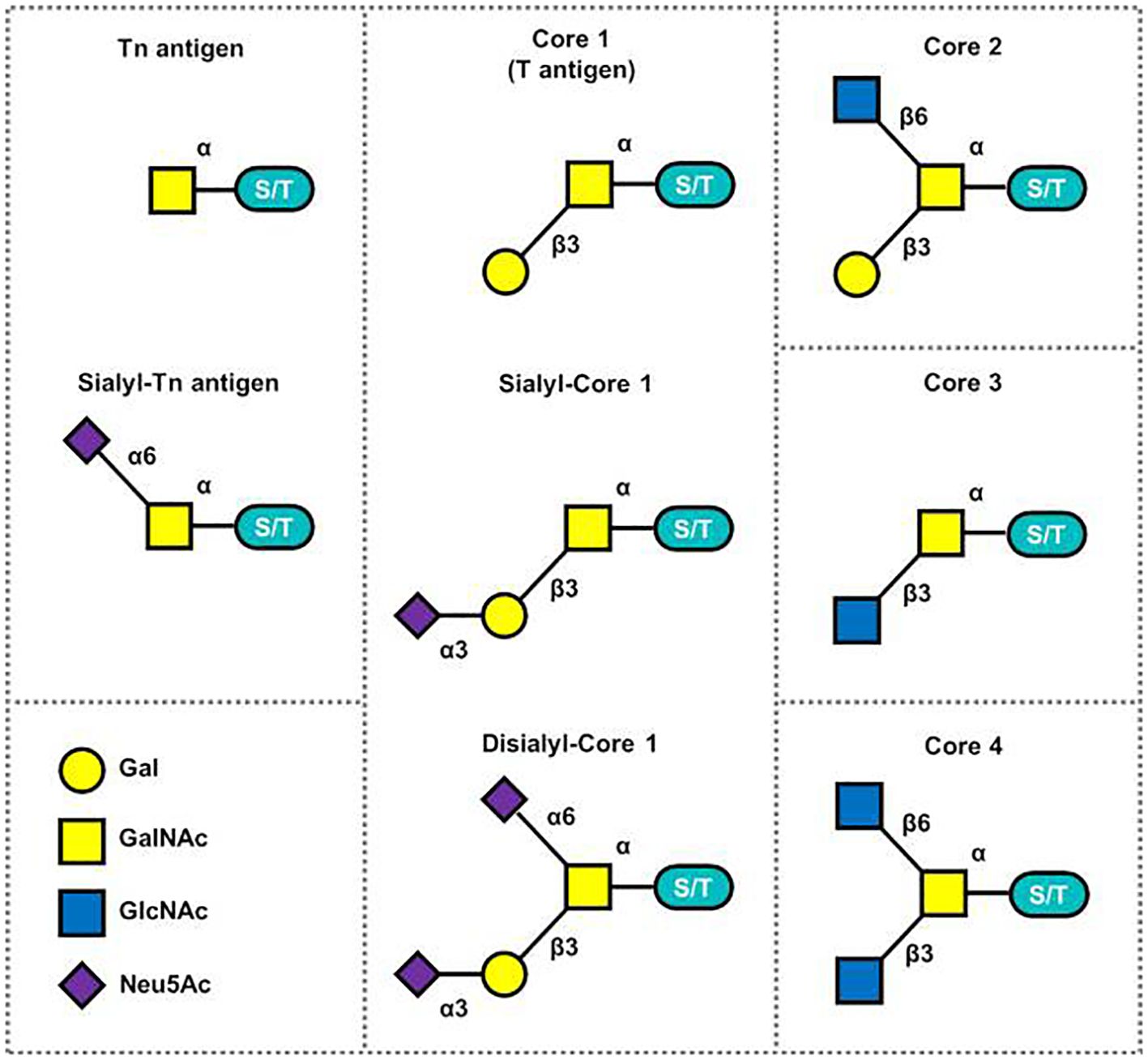
Figure 3 Mucin-type O-glycan core structures and key sialoglycoforms (51).
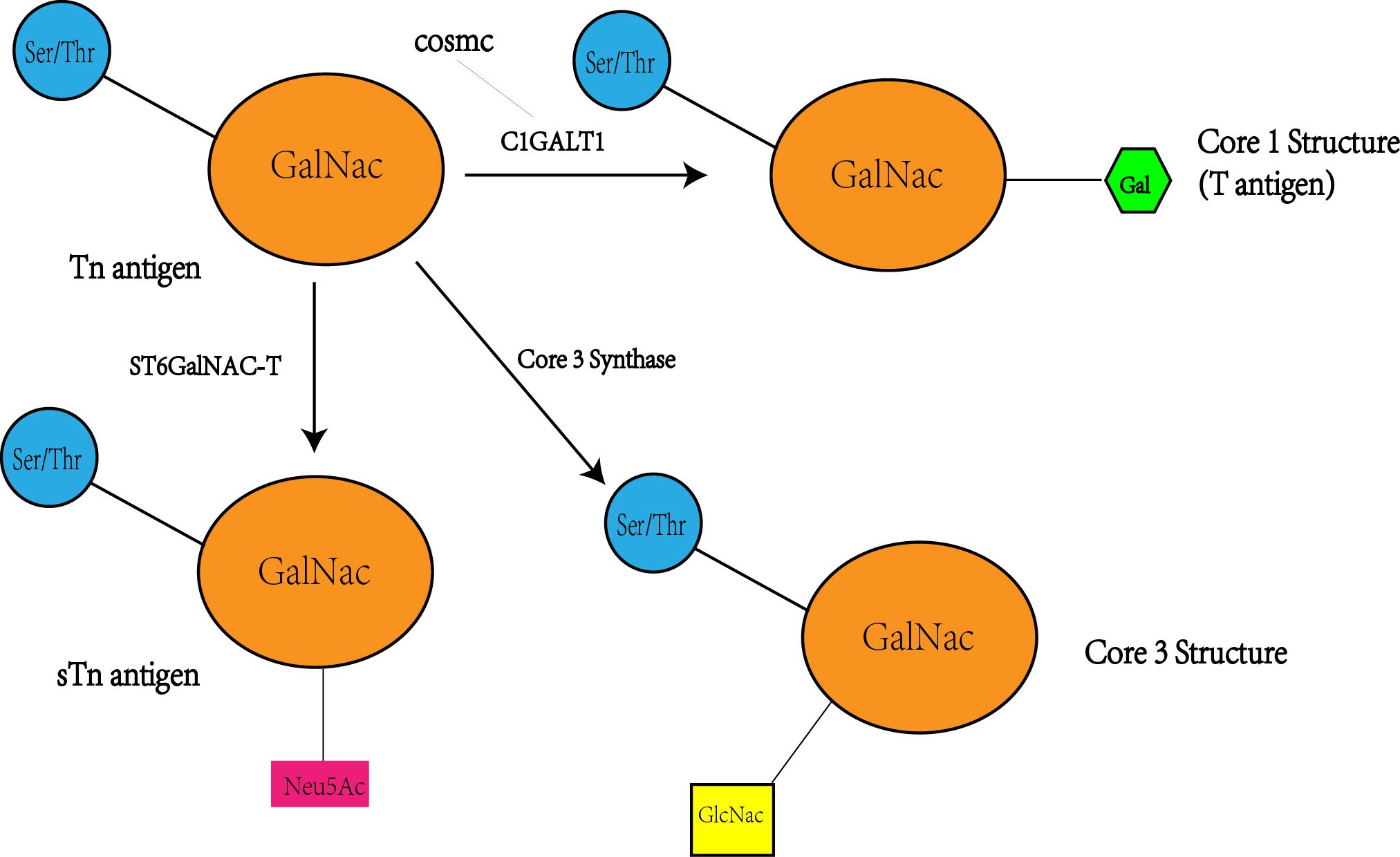
Figure 4 Tn antigen forms the T antigen i.e. core 1 structure under the action of C1GALT1, while the activity and level of CAGALT1 is regulated by COSMC. At the same time, Tn antigen can be converted to STn as well as core 3 structures catalyzed by the other two enzymes(ST6GalNAC; core 3 synthase i.e. β 3GnT6, C3GnT), so there may be a competitive relationship between these three enzymes, with a decrease in C1GALT1 levels accompanied by an increase in STn and core 3 structure levels.
3 C1GALT1 in colorectal cancer
Based on the information provided, it seems that C1GALT1 plays a crucial role in the glycosylation process, affecting the production of Tn antigen and the subsequent formation of downstream proteins like the core 1 structure. The mechanism of action of C1GALT1 is multifaceted and involves the regulation of target protein expression, phosphorylation, and localization, ultimately controlling various biological processes such as tumor proliferation, migration, and adhesion (25, 53, 54). In colorectal cancer cells, elevated T-synthase activity and overexpression of Cosmc and T synthase have been observed (55, 56). Recent studies have shown that SP cells isolated from human umbilical cord mesenchymal stem cells (hUCMSCs) and human placental mesenchymal stem cells (hPMSC) altered the O-glycosylation status by increasing O-glycosyltransferase activity, thereby inhibiting the proliferation and migration of Tn CRC cells and promoting apoptosis of Tn CRC cells (57). Based on these findings and previous research on cancer, several hypotheses regarding the mechanism of C1GALT1 action in colorectal cancer (CRC) have been proposed.
3.1 C1GALT1 and TACA
To begin with, in conjunction with the aforementioned information, Tumor-Associated Carbohydrate Antigens (TACA) such as Tn and sTn are produced during the O-glycosylation process, and C1GALT1 plays a significant role in this process. Notably, the detection of Tn and sTn antigens is prevalent in samples of colorectal cancer, linking their presence to the likelihood of cancer spread and a negative outlook for the patient (20, 58, 59). This connection implies that the appearance of Tn and sTn within cells could be one of the initial steps in the development of colorectal cancer (34). Moreover, the neo- or over-expression of Tn, sTn, and T antigens is not limited to colorectal cancer but occurs in many types of cancer, including gastric, colon, breast, lung, esophageal, prostate, and endometrial cancer (60, 61). This widespread occurrence underscores the significance of these antigens in cancer biology, particularly in promoting tumor metastasis. Approximately 86% of primary and metastatic human CRC tissues express Tn epitopes (62). Research has shown that the Tn antigen can trigger the Epithelial-Mesenchymal Transition (EMT) via the H-Ras gene within the Ras/MAPK signaling pathway (56). Furthermore, this activation leads to a decrease in epithelial cell markers, such as snail, and increases the metastatic capabilities of colon cancer cells. Additionally, the targeted suppression of the C1galt1c1 gene using CRISPR/Cas9 technology results in increased levels of Tn antigen on the surface of the colorectal cancer cell line MC38 (MC38-Tnhigh) (63). In a study of pancreatic cancer, by using the CRISPR/Cas9 system to disrupt C1GALT1 in human pancreatic ductal adenocarcinomas cells, the results showed enhanced invasiveness and metastatic ability of these cells and increased production of Tn (64). These observations collectively suggest that C1GALT1’s regulation of Tn and sTn antigen production plays a significant role in the development and progression of colorectal cancer by influencing its ability to spread.
3.2 C1GALT1 and core 3 synthase
The core 3 structure is a kind of O-glycans which plays an important role in the differentiation of gastrointestinal goblet cells and the formation of mucosal barrier β Mucin type O-glycans synthesized by 1,3-N acetylglucosamine transferase 6 (core 3 synthase, β 3GnT6, C3GnT) (65). The core 3 structure is synthesized by β1,3-N-acetylglucosaminyltransferase 6 (B3GNT6 or core 3 synthetase), which adds GlcNAc with a β1,3-linkage to the Tn antigen (GalNAc alpha-serine/threonine), and since core 1 synthetase makes use of the same 3′-position of GalNAc found in the Tn antigen, the synthesis of core 3 may compete with core 1 synthesis (64). Normal colon mucosa predominantly express core 3 O-glycans (50). The absence of O-glycans compromises the colonic mucus barrier, leading to inflammation through the activation of caspase 1-dependent inflammasomes in colonic epithelial cells, a process mediated by the microbiota (65). Another research has shown a novel mechanism by which mucin-type core 3 O-glycan influences the epithelial-mesenchymal transition (EMT) and mesenchymal-epithelial transition (MET) plasticity of colorectal cancer (CRC) cells through a MUC1/p53/miR-200c-dependent signaling cascade (66). An experiment has demonstrated that the inhibition of C1GALT1 is accompanied by an increase in the expression of sialic acid Tn and GSL-II binding (core 3 structure) in human colorectal cancer cells (67). Thus overexpression of C1GALT1 may reduce the core 3 structure, thereby inducing colorectal cancer. Additionally, decreased expression of core 3 synthase is associated with lymph node and distant organ metastasis, leading to poor prognosis in CRC patients (66). In a mouse experiment that showed a significant increase in the incidence of colon-related diseases with increasing age, the expression of C1GalT1 increased 1-fold, whereas the expression of core 2 β1,6-N-acetylglucosaminyltransferase (C2GnT) and core 3 β1,3-N-acetylglucosaminyltransferase (C3GnT) declined 2- to 6-fold and 2-fold, respectively (68). Therefore, the overexpression of C1GALT1, leading to a reduction in the quantity or activity of core 3 synthase and subsequent decrease in the core 3 structure, may contribute to the development of colon cancer.
3.3 C1GALT1 and Cosmc
Despite its significance, the precise structure of C1GALT1 and the mechanisms of substrate recognition and catalysis remain elusive (69), underscoring a gap in our understanding of how these antigens are produced at the molecular level. A key aspect of this process involves the unique molecular chaperone of C1GALT1, known as Cosmc, which is essential for the proper folding of T-synthase in the endoplasmic reticulum. The dysregulation of glycosyltransferases like C1GALT1, molecular chaperones like Cosmc, or the cellular environment can lead to the abnormal regulation of O-glycans, contributing to the development and progression of various cancers. A study revealed that the upregulation of C1GALT1 was accompanied by an increase in Cosmc levels in colorectal cancer cells (66). Further experiments demonstrated that C1GALT1 was absent in Cosmc-deficient cancer cells, consistent with previous reports indicating that the presence of T-synthase relies on intact Cosmc (56). It has also been observed that T-synthase activity and Cosmc, a crucial chaperone for its expression, were lower in Tn-positive CRC tissues compared to negative tissues (62). Others have proposed that the characteristic truncation of O-glycans found in pancreatic cancer and most epithelial cancers is not due to somatic mutations, but at least partially due to epigenetic silencing of COSMC companion genes caused by promoter hypermethylation (52). While, Sun (34) et al. have found that LOH that is a common mechanism of loss of gene function in tumorigenesis occurs in Cosmc, but not C1GALT1, through studies of CRC cells. The expression and mutation of Cosmc significantly impact C1GALT1 activity, levels, and Tn antigen expression, thereby playing a crucial role in the development of colorectal cancer.
3.4 Downstream regulators of C1GALT1
C1GALT1, a key player in colon cancer pathogenesis, significantly influences the behavior and properties of cancer cells. This enzyme’s overexpression alters O-glycans on Fibroblast Growth Factor Receptor 2 (FGFR2), a receptor tyrosine kinase overexpressed in colorectal cancer (53). FGFR2 is crucial for cellular processes like proliferation, survival, migration, and differentiation (70, 71). The modification of FGFR2 by C1GALT1, as evidenced by the presence of sTn on FGFR2, enhances its phosphorylation, promoting invasive behavior and cancer stem-like properties in colon cancer cells (53, 72–76). Furthermore, C1GALT1’s role extends to the epithelial-mesenchymal transition (EMT) in cancer cells. A deficiency in C1GALT1 leads to the classical EMT profile, characterized by a reduction in E-cadherin (an epithelial marker) and an increase in mesenchymal markers like snail and fibronectin. This change in cellular markers indicates a transformation in the cancer cells’ behavior and properties (77). Additionally, C1GALT1 suppression impacts tumor cell interactions and activities. It notably reduces galectin-3-mediated tumor cell-cell interaction and the promotion of tumor cell activities by galectin-3 (69). A study on colon cancer cells found that the expression of silyl-Tn was associated with an increase in the α2,6-carbamoyltransferase gene (ST6GALNAC1) and a decrease in the core 1 synthase gene (C1GALT1) in LS174T cells, by qRT-PCR (78). A recent study on endometrial cancer showed that low expression of C1GALT1 induced overexpression of ANXA1 in ECC-1 cells, which were characterized by higher proliferation, invasion, migration, colony formation and angiogenesis (79). Besides, C1GALT1 is able to modify O-linked glycosylation on integrin α5, thereby modulating activation of the PI3K/AKT pathway in gastric cancer cells (80). Also in pancreatic cancer, C1GALT1 knockdown significantly inhibited cell adhesion to the extracellular matrix (ECM), which was associated with a decrease in FAK phosphorylation at Y397/Y925 as well as changes in O-glycans on integrins (including β1, αv and α5 subunits) (81). In addition, C1GALT1 affects the migratory ability, proliferation and colony formation of bladder cancer cells through a mechanism of miR-1-3p/cHP1BP3 axis deregulation and shows tumor suppressor activity in bladder cancer cells (82). Recent research has unveiled that knockout of the Zn2+-transporter SLC39A9 (ZIP9), alongside the well-described targets C1GALT1 (C1GalT1) and its molecular chaperone, C1GALT1C1 (COSMC), results in surface-expression of cancer-associated O-glycans (83). In gastric cancer, C1GALT1 promotes EPHA2 phosphorylation and enhances soluble Ephrin A1-mediated migration mainly by modifying the O-glycosylation of EPHA2, thereby affecting the cell invasiveness of gastric cancer cells (84). This further underscores C1GALT1’s significant role in the progression and characteristics of epithelial cancers, including colon cancer.
3.5 C1GALT1 and MUC1
Due to the high concentration of O-linked GalNAc on mucin proteins, further refinement produces what is commonly referred to as mucin-type O-glycans. The exposed Tn antigen is the ligand of Ca2+dependent C-type lectin receptor MGL (macrophage galactose type lectin/CD301/CLEC10A) (85), MGL specifically recognizes tumor derived mucin MUC1 by binding to Tn antigen (86). Mucin 1 (MUC1) is a single channel type I transmembrane protein with a highly glycosylated extracellular domain, which is usually located at the root tip edge of epithelial cells and plays a protective role in lower epithelial cells (87). Altered glycosylation of the oncoprotein MUC1 commonly occurs in chronic inflammation, including ulcerative colitis, and this aberrantly glycosylated MUC1 promotes cancer development and progression (88). While, MUC1 deficiency has been shown to suppress inflammation, inhibit tumor progression, increase the abundance of CD8 T-lymphocytes, and decrease the abundance of macrophages in colon tumors (89). In various epithelial cancers MUC1 has abnormal glycosylation and overexpression (90, 91). Increased expression of MUC1 in CRC is associated with worse prognosis metastasis (87, 92). Besides, MUC1 participates in complex immune process and has immunomodulatory effect (93, 94).And, the latest research has found that crosstalk between macrophages and colonocytes increasing MUC1-sTn expression (88). The expression of T, sTn on tumor mucins such as MUC1 which plays as various selectins of ligands, caused by abnormal glycosylation promotes metastasis and spread (95). Mucins are carriers of selectin ligands, facilitate metastasis and spread by forming aggregates with cells which express selectins on their surface (96–98). Cancer cells use this ability of mucin to escape immune surveillance (95). Therefore, mucins play an important role in cancer metastasis and diffusion.
The N-terminal of MUC1 contains VNTR fragment, which contains serine and threonine residues and provides a number of O-glycosylation sites (99), including T and sTn antigens (100). In MUC1 with insufficient glycosylation, the VNTR region containing exposed cryptic peptide epitopes has been proved to elicit strong humoral- and cell-mediated immune responses (95). Based on this theory, in recent years, many studies have found the target of MUC1 anti-tumor vaccine, and the results showed that the survival rate was improved (101) and the metastatic foci were reduced in the mouse model. In the experiment of human colorectal cancer cells, anti MUC1 shows potential for colorectal cancer treatment (102, 103). Targeting MUC1-C to inhibit the AKT-S6K1-elF4A pathway regulates TIGAR translation in colorectal cancer and inhibits the growth of colon cancer cells in vitro (104). Glycosylation of MUC1 depends on the biomolecules it recognizes, such as, for example, GalNAc transferase, visfatin lectin, antibodies and other glycosyltransferases (105), while a research found that anti MUC1 treatment can inhibit C1GALT1 at protein level (106). In other words, the impact of C1GALT1 on MUC1 glycosylation has a certain influence on the development of colorectal cancer, although the specific mechanism is not yet fully understood. It has been found in the study of esophageal cancer, another epithelial cell carcinoma that the expression level of C1GALT1 was positively correlated with MUC1 O- glycosylation, and the co expression of MUC1 and C1GALT1 was negatively correlated with survival rate (26). Therefore, it is plausible to hypothesize that a similar pathway involving MUC1 may be involved in the development of colorectal cancer. However, further studies are still required to elucidate the exact mechanisms underlying this relationship.
4 Summary
The prospect of C1GALT1 in colorectal cancer (CRC) is multifaceted, mainly in terms of its key role in the process of tumorigenesis and progression as well as its potential as a potential therapeutic target. C1GALT1 produces tumor-associated carbohydrate antigens (TACA), such as Tn and sTn antigens, through the process of O-glycosylation, and these antigens have been associated with the spread of colorectal cancer and poor patient prognosis. The O-glycosylation of the core 3 structure is essential for the differentiation of gastrointestinal tract cup cells and the formation of the mucosal barrier.Regulation of core 3 structure expression by C1GALT1 may affect the development of colorectal cancer, and its overexpression may reduce the core 3 structure, which can induce cancer. Cosmc acts as a molecular chaperone for C1GALT1 and is essential for the correct folding of T-synthase in the endoplasmic reticulum.Expression and mutation of Cosmc significantly affects the activity of C1GALT1 and the expression of Tn antigens, emphasizing its role in CRC. C1GALT1 affects the behavior of cancer cells by altering the O-glycosylation on FGFR2, which promotes cancer cell invasiveness and stem cell-like properties.C1GALT1 also affects the epithelial-mesenchymal transition (EMT) of cancer cells, indicating its important role in cancer progression. Aberrant glycosylation of MUC1 plays a role in cancer development and progression.The effect of C1GALT1 on MUC1 glycosylation plays a role in CRC development, and cancer therapies targeting MUC1 show potential, suggesting a potential effect of C1GALT1 on MUC1 glycosylation. The specific interactions and regulatory mechanisms between C1GALT1 and TACA, core 3 synthase, Cosmc, downstream regulators, and MUC1 were further explored to better understand its role in CRC. Based on the key role of C1GALT1 in CRC, the development of inhibitors or activators targeting its activity, expression or its interaction with other molecules as a new therapeutic strategy. Considering the interactions of C1GALT1 with multiple molecules and pathways, exploring combinatorial therapeutic strategies that combine treatments targeting C1GALT1 with other therapeutic approaches (e.g., chemotherapy, immunotherapy) to improve therapeutic efficacy. The pathway described above is specifically shown in Figure 5. This review highlights the potential mechanisms by which C1GALT1 contributes to the carcinogenesis of CRC. It holds promise as a specific therapeutic target for CRC. Furthermore, the precise mechanism of C1GALT1 in CRC has yet to be fully elucidated, presenting strong prospects for further research. The next step could be to develop inhibitors targeting C1GALT1 activity or expression, and assess their effects on CRC cell proliferation and invasion through in vitro and in vivo experiments. Also explore the molecules and pathways that interact with C1GALT1 (including COSMC and Core 3 Synthase, etc.) and develop corresponding activators or inhibitors to modulate its role in CRC. This may be a promising research direction.
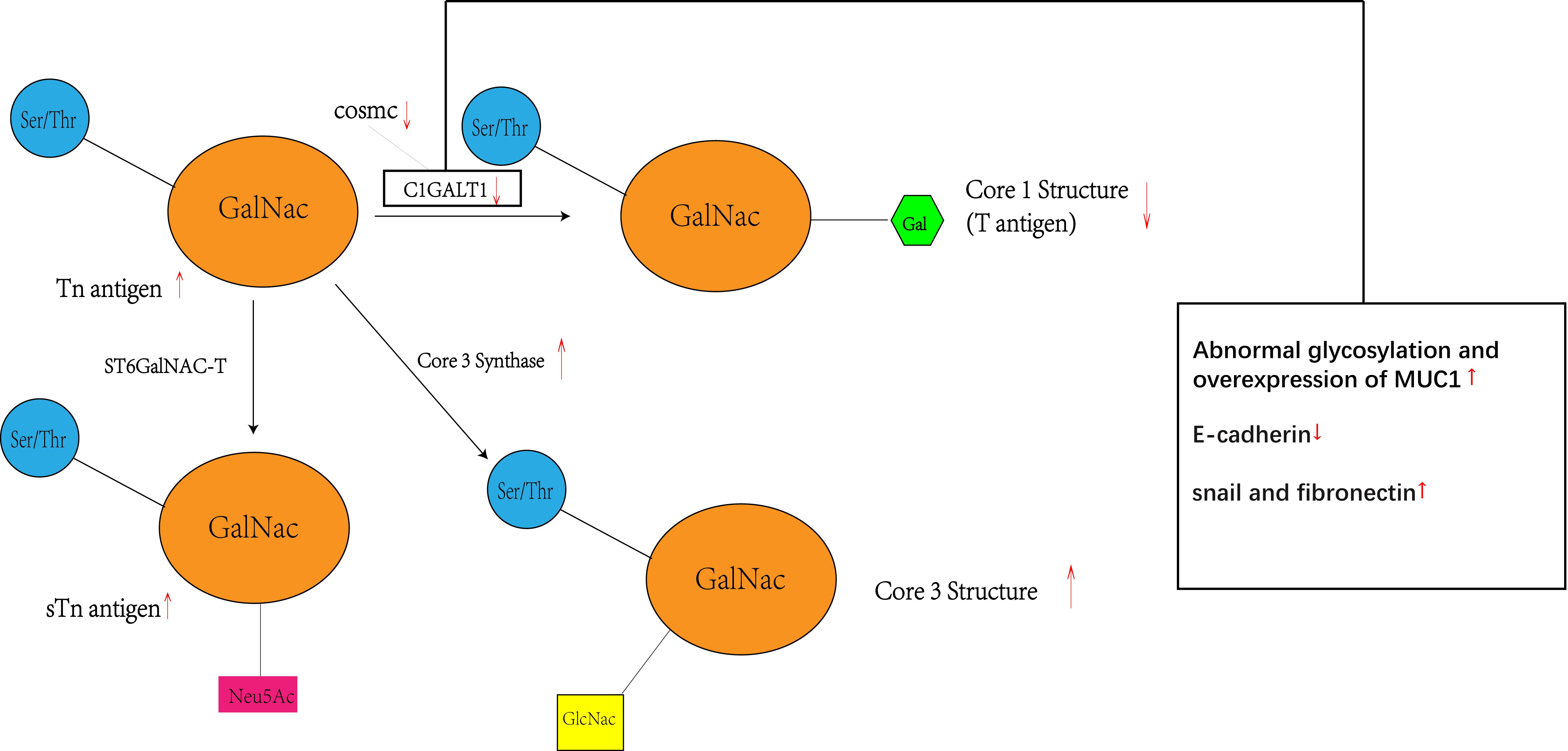
Figure 5 Inhibition of C1GALT1 leads to elevated Tn antigen, and due to competition, inhibition of C1GALT1 leads to a relative dominance of ST6GalNAC and core 3 synthase, resulting in elevated levels of sTn antigen and core 3 structure and decreased levels of core 1 structure. At the same time C1GALT1 deficiency directly induces the classical EMT signature of cancer cells, i.e., a marked decrease in the typical epithelial cell marker E-cadherin and enhanced expression of isochronous stromal markers, including snail and fibronectin. Meanwhile C1GALT1 inhibition induces abnormal glycosylation and overexpression of MUC1.
Author contributions
HT: Writing – review & editing, Supervision, Funding acquisition, Conceptualization. J-L Yu: Data curation, Writing – original draft. XC: Writing – review & editing, Data curation. QG: Writing – review & editing, Formal analysis. JL: Writing – review & editing, Formal analysis. YL: Writing – review & editing, Resources.
Funding
The author(s) declare financial support was received for the research, authorship, and/or publication of this article. This work was supported by the Natural Science Foundation of Liaoning Province (2020-MS-327) and the Health Commission of Shenyang (2021-wjkt-009).
Conflict of interest
The authors declare that the research was conducted in the absence of any commercial or financial relationships that could be construed as a potential conflict of interest.
Publisher’s note
All claims expressed in this article are solely those of the authors and do not necessarily represent those of their affiliated organizations, or those of the publisher, the editors and the reviewers. Any product that may be evaluated in this article, or claim that may be made by its manufacturer, is not guaranteed or endorsed by the publisher.
References
1. Dekker E, Tanis PJ, Vleugels JLA, Kasi PM, Wallace MB. Colorectal cancer. Lancet. (2019) 394:1467–80. doi: 10.1016/S0140-6736(19)32319-0
2. Arnold M, Sierra MS, Laversanne M, Soerjomataram I, Jemal A, Bray F. Global patterns and trends in colorectal cancer incidence and mortality. GUT. (2017) 66:683–91. doi: 10.1136/gutjnl-2015-310912
3. Araghi M, Soerjomataram I, Jenkins M, Brierley J, Morris E, Bray F, et al. Global trends in colorectal cancer mortality: projections to the year 2035. Int J Cancer. (2019) 144:2992–3000. doi: 10.1002/ijc.32055
4. Kasi PM, Shahjehan F, Cochuyt JJ, Li Z, Colibaseanu DT, Merchea A. Rising proportion of young individuals with rectal and colon cancer. Clin Colorectal Cancer. (2019) 18:e87–95. doi: 10.1016/j.clcc.2018.10.002
5. Read B, Sylla P. Aggressive colorectal cancer in the young. Clin Colon Rectal Surg. (2020) 33:298–304. doi: 10.1055/s-0040-1713747
6. Baidoun F, Elshiwy K, Elkeraie Y, Merjaneh Z, Khoudari G, Sarmini MT, et al. Colorectal cancer epidemiology: recent trends and impact on outcomes. Curr Drug Targets. (2021) 22:998–1009. doi: 10.2174/1389450121999201117115717
7. Brenner H, Kloor M, Pox CP. Colorectal cancer. Lancet. (2014) 383:1490–502. doi: 10.1016/S0140-6736(13)61649-9
8. Onyoh EF, Hsu WF, Chang LC, Lee YC, Wu MS, Chiu HM. The rise of colorectal cancer in Asia: epidemiology, screening, and management. Curr Gastroenterol Rep. (2019) 21:36. doi: 10.1007/s11894-019-0703-8
9. McQuade RM, Stojanovska V, Bornstein JC, Nurgali K. Colorectal cancer chemotherapy: the evolution of treatment and new approaches. Curr Med Chem. (2017) 24:1537–57. doi: 10.2174/0929867324666170111152436
10. Peixoto A, Relvas-Santos M, Azevedo R, Santos LL, Ferreira JA. Protein glycosylation and tumor microenvironment alterations driving cancer hallmarks. Front Oncol. (2019) 9:380. doi: 10.3389/fonc.2019.00380
11. Thomas D, Rathinavel AK, Radhakrishnan P. Altered glycosylation in cancer: A promising target for biomarkers and therapeutics. Biochim Biophys Acta Rev Cancer. (2021) 1875:188464. doi: 10.1016/j.bbcan.2020.188464
13. Schjoldager KT, Narimatsu Y, Joshi HJ, Clausen H. Global view of human protein glycosylation pathways and functions. Nat Rev Mol Cell Biol. (2020) 21:729–49. doi: 10.1038/s41580-020-00294-x
14. Tarp MA, Clausen H. Mucin-type O-glycosylation and its potential use in drug and vaccine development. Biochim Biophys Acta. (2008) 1780:546–63. doi: 10.1016/j.bbagen.2007.09.010
15. Higel F, Seidl A, Sorgel F, Friess W. N-glycosylation heterogeneity and the influence on structure, function and pharmacokinetics of monoclonal antibodies and Fc fusion proteins. Eur J Pharm Biopharm. (2016) 100:94–100. doi: 10.1016/j.ejpb.2016.01.005
16. Shan A, Lu J, Xu Z, Li X, Xu Y, Li W, et al. Polypeptide N-acetylgalactosaminyltransferase 18 non-catalytically regulates the ER homeostasis and O-glycosylation. Biochim Biophys Acta (BBA) - Gen Subj. (2019) 1863:870–82. doi: 10.1016/j.bbagen.2019.01.009
17. Wulff-Fuentes E, Berendt RR, Massman L, Danner L, Malard F, Vora J, et al. The human O-GlcNAcome database and meta-analysis. Sci Data. (2021) 8:25. doi: 10.1038/s41597-021-00810-4
18. Bennett EP, Mandel U, Clausen H, Gerken TA, Fritz TA, Tabak LA. Control of mucin-type O-glycosylation: a classification of the polypeptide GalNAc-transferase gene family. Glycobiology. (2012) 22:736–56. doi: 10.1093/glycob/cwr182
19. Wu YM, Liu CH, Huang MJ, Lai HS, Lee PH, Hu RH, et al. C1GALT1 enhances proliferation of hepatocellular carcinoma cells via modulating MET glycosylation and dimerization. Cancer Res. (2013) 73:5580–90. doi: 10.1158/0008-5472.CAN-13-0869
20. Liu CH, Hu RH, Huang MJ, Lai IR, Chen CH, Lai HS, et al. C1GALT1 promotes invasive phenotypes of hepatocellular carcinoma cells by modulating integrin beta1 glycosylation and activity. PloS One. (2014) 9:e94995. doi: 10.1371/journal.pone.0094995
21. Liu YY, Liu HY, Yu TJ, Lu Q, Zhang FL, Liu GY, et al. O-GlcNAcylation of MORC2 at threonine 556 by OGT couples TGF-beta signaling to breast cancer progression. Cell Death Differ. (2022) 29:861–73. doi: 10.1038/s41418-021-00901-0
22. Zhu Q, Zhou H, Wu L, Lai Z, Geng D, Yang W, et al. O-GlcNAcylation promotes pancreatic tumor growth by regulating malate dehydrogenase 1. Nat Chem Biol. (2022) 18:1087–95. doi: 10.1038/s41589-022-01085-5
23. He X, Li Y, Chen Q, Zheng L, Lou J, Lin C, et al. O-GlcNAcylation and stablization of SIRT7 promote pancreatic cancer progression by blocking the SIRT7-REGgamma interaction. Cell Death Differ. (2022) 29:1970–81. doi: 10.1038/s41418-022-00984-3
24. Liu F, Fu J, Bergstrom K, Shan X, McDaniel JM, McGee S, et al. Core 1-derived mucin-type O-glycosylation protects against spontaneous gastritis and gastric cancer. J Exp Med. (2020) 217. doi: 10.1084/jem.20182325
25. Tan Z, Jiang Y, Liang L, Wu J, Cao L, Zhou X, et al. Dysregulation and prometastatic function of glycosyltransferase C1GALT1 modulated by cHP1BP3/ miR-1-3p axis in bladder cancer. J Exp Clin Cancer Res. (2022) 41:228. doi: 10.1186/s13046-022-02438-7
26. Wang Y, Liao X, Ye Q, Huang L. Clinic implication of MUC1 O-glycosylation and C1GALT1 in esophagus squamous cell carcinoma. Sci China Life Sci. (2018) 61:1389–95. doi: 10.1007/s11427-017-9345-7
27. Khiaowichit J, Talabnin C, Dechsukhum C, Silsirivanit A, Talabnin K. Down-regulation of C1GALT1 enhances the progression of cholangiocarcinoma through activation of AKT/ERK signaling pathways. Life (Basel). (2022) 12. doi: 10.3390/life12020174
28. Holst S, Wuhrer M, Rombouts Y. Glycosylation characteristics of colorectal cancer. Adv Cancer Res. (2015) 126:203–56. doi: 10.1016/bs.acr.2014.11.004
29. Fernandez-Ponce C, Geribaldi-Doldan N, Sanchez-Gomar I, Quiroz RN, Ibarra LA, Escorcia LG, et al. The role of glycosyltransferases in colorectal cancer. Int J Mol Sci. (2021) 22. doi: 10.3390/ijms22115822
30. Wang B, Ye Y, Yang X, Liu B, Wang Z, Chen S, et al. SIRT2-dependent IDH1 deacetylation inhibits colorectal cancer and liver metastases. EMBO Rep. (2020) 21:e48183. doi: 10.15252/embr.201948183
31. Hou JY, Cao J, Gao LJ, Zhang FP, Shen J, Zhou L, et al. Upregulation of alpha enolase (ENO1) crotonylation in colorectal cancer and its promoting effect on cancer cell metastasis. Biochem Biophys Res Commun. (2021) 578:77–83. doi: 10.1016/j.bbrc.2021.09.027
32. Ferreira JA, Magalhaes A, Gomes J, Peixoto A, Gaiteiro C, Fernandes E, et al. Protein glycosylation in gastric and colorectal cancers: Toward cancer detection and targeted therapeutics. Cancer Lett. (2017) 387:32–45. doi: 10.1016/j.canlet.2016.01.044
33. Xia T, Xiang T, Xie H. Update on the role of C1GALT1 in cancer. Oncol Lett. (2022) 23:97. doi: 10.3892/ol
34. Sun X, Ju T, Cummings RD. Differential expression of Cosmc, T-synthase and mucins in Tn-positive colorectal cancers. BMC Cancer. (2018) 18:827. doi: 10.1186/s12885-018-4708-8
35. Petrosyan A, Ali MF, Cheng PW. Glycosyltransferase-specific golgi-targeting mechanisms. J Biol Chem. (2012) 287:37621–7. doi: 10.1074/jbc.C112.403006
36. Gonzalez-Ramirez AM, Grosso AS, Yang Z, Companon I, Coelho H, Narimatsu Y, et al. Structural basis for the synthesis of the core 1 structure by C1GalT1. Nat Commun. (2022) 13:2398. doi: 10.1038/s41467-022-29833-0
37. Brockhausen I, Schachter H, Stanley P, Varki A, Cummings RD, Esko JD, et al. O-GalNAc Glycans. Essentials of glycobiology. Cold Spring Harbor (NY (2022). p. 117–28.
38. Zhang L, Ten Hagen KG. Pleiotropic effects of O-glycosylation in colon cancer. J Biol Chem. (2018) 293:1315–6. doi: 10.1074/jbc.H117.812826
39. Springer GF. T. and Tn, general carcinoma autoantigens. Science. (1984) 224:1198–206. doi: 10.1126/science.6729450
40. Sewell R, Backstrom M, Dalziel M, Gschmeissner S, Karlsson H, Noll T, et al. The ST6GalNAc-I sialyltransferase localizes throughout the Golgi and is responsible for the synthesis of the tumor-associated sialyl-Tn O-glycan in human breast cancer. J Biol Chem. (2006) 281:3586–94. doi: 10.1074/jbc.M511826200
41. Crew VK, Singleton BK, Green C, Parsons SF, Daniels G, Anstee DJ. New mutations in C1GALT1C1 in individuals with Tn positive phenotype. Br J Haematol. (2008) 142:657–67. doi: 10.1111/j.1365-2141.2008.07215.x
42. Yue S, Wang X, Ge W, Li J, Yang C, Zhou Z, et al. Deciphering protein O-GalNAcylation: method development and disease implication. ACS Omega. (2023) 8:19223–36. doi: 10.1021/acsomega.3c01653
43. Ju T, Otto VI, Cummings RD. The Tn antigen-structural simplicity and biological complexity. Angewandte Chemie Int Edition. (2011) 50:1770–91. doi: 10.1002/anie.201002313
44. Ju T, Lanneau GS, Gautam T, Wang Y, Xia B, Stowell SR, et al. Human tumor antigens Tn and sialyl Tn arise from mutations in Cosmc. Cancer Res. (2008) 68:1636–46. doi: 10.1158/0008-5472.CAN-07-2345
45. Zeng J, Mi R, Wang Y, Li Y, Lin L, Yao B, et al. Promoters of human cosmc and T-synthase genes are similar in structure, yet different in epigenetic regulation. J Biol Chem. (2015) 290:19018–33. doi: 10.1074/jbc.M115.654244
46. Wan Y, Yu LG. Expression and impact of C1GalT1 in cancer development and progression. Cancers (Basel). (2021) 13. doi: 10.3390/cancers13246305
47. Beckwith DM, Cudic M. Tumor-associated O-glycans of MUC1: Carriers of the glyco-code and targets for cancer vaccine design. Semin Immunol. (2020) 47:101389. doi: 10.1016/j.smim.2020.101389
48. Kurosawa N, Kojima N, Inoue M, Hamamoto T, Tsuji S. Cloning and expression of Gal beta 1,3GalNAc-specific GalNAc alpha 2,6-sialyltransferase. J Biol Chem. (1994) 269:19048–53. doi: 10.1016/S0021-9258(17)32272-X
49. Iwai T, Inaba N, Naundorf A, Zhang Y, Gotoh M, Iwasaki H, et al. Molecular cloning and characterization of a novel UDP-GlcNAc:GalNAc-peptide beta1,3-N-acetylglucosaminyltransferase (beta 3Gn-T6), an enzyme synthesizing the core 3 structure of O-glycans. J Biol Chem. (2002) 277:12802–9. doi: 10.1074/jbc.M112457200
50. Madunić K, Mayboroda OA, Zhang T, Weber J, Boons GJ, Morreau H, et al. Specific (sialyl-)Lewis core 2 O-glycans differentiate colorectal cancer from healthy colon epithelium. Theranostics. (2022) 12(10):4498–512. doi: 10.7150/thno.72818
51. Thompson N, Wakarchuk W. O-glycosylation and its role in therapeutic proteins. Biosci Rep. (2022) 42. doi: 10.1042/BSR20220094
52. Radhakrishnan P, Dabelsteen S, Madsen FB, Francavilla C, Kopp KL, Steentoft C, et al. Immature truncated O-glycophenotype of cancer directly induces oncogenic features. Proc Natl Acad Sci U.S.A. (2014) 111:E4066–75. doi: 10.1073/pnas.1406619111
53. Hung JS, Huang J, Lin YC, Huang MJ, Lee PH, Lai HS, et al. C1GALT1 overexpression promotes the invasive behavior of colon cancer cells through modifying O-glycosylation of FGFR2. Oncotarget. (2014) 5:2096–106. doi: 10.18632/oncotarget.v5i8
54. Tsai C-H, Tzeng S-F, Chao T-K, Tsai C-Y, Yang Y-C, Lee M-T, et al. Metastatic progression of prostate cancer is mediated by autonomous binding of galectin-4-O-Glycan to cancer cells. Cancer Res. (2016) 76:5756–67. doi: 10.1158/0008-5472.CAN-16-0641
55. Gao T, Du T, Hu X, Dong X, Li L, Wang Y, et al. Cosmc overexpression enhances Malignancies in human colon cancer. J Cell Mol Med. (2020) 24:362–70. doi: 10.1111/jcmm.14740
56. Liu Z, Liu J, Dong X, Hu X, Jiang Y, Li L, et al. Tn antigen promotes human colorectal cancer metastasis via H-Ras mediated epithelial-mesenchymal transition activation. J Cell Mol Med. (2019) 23:2083–92. doi: 10.1111/jcmm.14117
57. Hu W, Ding R, Wang M, Huang P, Wei X, Hu X, et al. Side population cells derived from hUCMSCs and hPMSCs could inhibit the Malignant behaviors of Tn(+) colorectal cancer cells from modifying their O-glycosylation status. Stem Cell Res Ther. (2023) 14:145. doi: 10.1186/s13287-023-03334-3
58. Konno A, Hoshino Y, Terashima S, Motoki R, Kawaguchi T. Carbohydrate expression profile of colorectal cancer cells is relevant to metastatic pattern and prognosis. Clin Exp Metastasis. (2002) 19:61–70. doi: 10.1023/A:1013879702702
59. Sletmoen M, Gerken TA, Stokke BT, Burchell J, Brewer CF. Tn and STn are members of a family of carbohydrate tumor antigens that possess carbohydrate-carbohydrate interactions. Glycobiology. (2018) 28:437–42. doi: 10.1093/glycob/cwy032
60. Rajesh C, Radhakrishnan P. The (Sialyl) Tn antigen: Contributions to immunosuppression in gastrointestinal cancers. Front Oncol. (2023) 12. doi: 10.3389/fonc.2022.1093496
61. Fu C, Zhao H, Wang Y, Cai H, Xiao Y, Zeng Y, et al. Tumor-associated antigens: Tn antigen, sTn antigen, and T antigen. Hla. (2016) 88:275–86. doi: 10.1111/tan.12900
62. Jiang Y, Liu Z, Xu F, Dong X, Cheng Y, Hu Y, et al. Aberrant O-glycosylation contributes to tumorigenesis in human colorectal cancer. J Cell Mol Med. (2018) 22:4875–85. doi: 10.1111/jcmm.13752
63. Cornelissen LAM, Blanas A, Zaal A, van der Horst JC, Kruijssen LJW, O'Toole T, et al. Tn antigen expression contributes to an immune suppressive microenvironment and drives tumor growth in colorectal cancer. Front Oncol. (2020) 10:1622. doi: 10.3389/fonc.2020.01622
64. Chugh S, Barkeer S, Rachagani S, Nimmakayala RK, Perumal N, Pothuraju R, et al. Disruption of C1galt1 gene promotes development and metastasis of pancreatic adenocarcinomas in mice. Gastroenterology. (2018) 155:1608–24. doi: 10.1053/j.gastro.2018.08.007
65. Boottanun P, Ino Y, Shimada K, Hiraoka N, Angata K, Narimatsu H. Association between the expression of core 3 synthase and survival outcomes of patients with cholangiocarcinoma. Oncol Lett. (2021) 22:760. doi: 10.3892/ol
66. Bergstrom K, Liu X, Zhao Y, Gao N, Wu Q, Song K, et al. Defective intestinal mucin-type O-Glycosylation causes spontaneous colitis-associated cancer in mice. Gastroenterology. (2016) 151:152–64.e11.
67. Ye J, Wei X, Shang Y, Pan Q, Yang M, Tian Y, et al. Core 3 mucin-type O-glycan restoration in colorectal cancer cells promotes MUC1/p53/miR-200c-dependent epithelial identity. Oncogene. (2017) 36:6391–407. doi: 10.1038/onc.2017.241
68. Barrow H, Tam B, Duckworth CA, Rhodes JM, Yu LG. Suppression of core 1 Gal-transferase is associated with reduction of TF and reciprocal increase of Tn, sialyl-Tn and Core 3 glycans in human colon cancer cells. PloS One. (2013) 8:e59792. doi: 10.1371/journal.pone.0059792
69. Sang X, Wang Q, Ning Y, Wang H, Zhang R, Li Y, et al. Age-Related Mucus Barrier Dysfunction in Mice Is Related to the Changes in Muc2 Mucin in the Colon. Nutrients. (2023) 15(8):1830. doi: 10.3390/nu15081830
70. Wan Y, Adair K, Herrmann A, Shan X, Xia L, Duckworth CA, et al. C1GalT1 expression reciprocally controls tumour cell-cell and tumour-macrophage interactions mediated by galectin-3 and MGL with double impact on cancer development and progression. Cell Death Dis. (2023) 14. doi: 10.1038/s41419-023-06082-7
71. Yasui H, Takeno A, Hara H, Imamura H, Akamatsu H, Fujitani K, et al. Prospective analysis of the expression status of FGFR2 and HER2 in colorectal and gastric cancer populations: DS-Screen Study. Int J Colorectal Dis. (2022) 37:1393–402. doi: 10.1007/s00384-022-04162-2
72. Liu Y, Liu Y, Deng J, Li W, Nie X. Fibroblast growth factor in diabetic foot ulcer: progress and therapeutic prospects. Front Endocrinol (Lausanne). (2021) 12:744868. doi: 10.3389/fendo.2021.744868
73. Zingg D, Bhin J, Yemelyanenko J, Kas SM, Rolfs F, Lutz C, et al. Truncated FGFR2 is a clinically actionable oncogene in multiple cancers. Nature. (2022) 608:609–17. doi: 10.1038/s41586-022-05066-5
74. Mathur A, Ware C, Davis L, Gazdar A, Pan BS, Lutterbach B. FGFR2 is amplified in the NCI-H716 colorectal cancer cell line and is required for growth and survival. PloS One. (2014) 9:e98515. doi: 10.1371/journal.pone.0098515
75. Matsuda Y, Ueda J, Ishiwata T. Fibroblast growth factor receptor 2: expression, roles, and potential as a novel molecular target for colorectal cancer. Patholog Res Int. (2012) 2012:574768. doi: 10.1155/2012/574768
76. Li P, Huang T, Zou Q, Liu D, Wang Y, Tan X, et al. FGFR2 promotes expression of PD-L1 in colorectal cancer via the JAK/STAT3 signaling pathway. J Immunol. (2019) 202:3065–75. doi: 10.4049/jimmunol.1801199
77. Katoh M. FGFR inhibitors: Effects on cancer cells, tumor microenvironment and whole-body homeostasis (Review). Int J Mol Med. (2016) 38:3–15. doi: 10.3892/ijmm.2016.2620
78. Dong X, Jiang Y, Liu J, Liu Z, Gao T, An G, et al. T-synthase deficiency enhances oncogenic features in human colorectal cancer cells via activation of epithelial-mesenchymal transition. BioMed Res Int. (2018) 2018:9532389. doi: 10.1155/2018/9532389
79. Chik JH, Zhou J, Moh ES, Christopherson R, Clarke SJ, Molloy MP, et al. Comprehensive glycomics comparison between colon cancer cell cultures and tumours: Implications for biomarker studies. J Proteomics (2014) 108:146–62. doi: 10.1016/j.jprot.2014.05.002
80. Montero-Calle A, López-Janeiro Á, Mendes ML, Perez-Hernandez D, Echevarría I, Ruz-Caracuel I, et al. In-depth quantitative proteomics analysis revealed C1GALT1 depletion in ECC-1 cells mimics an aggressive endometrial cancer phenotype observed in cancer patients with low C1GALT1 expression. Cell Oncol (2023) 46(3):697–715. doi: 10.1007/s13402-023-00778-w
81. Dong X, Chen C, Deng X, Liu Y, Duan Q, Peng Z, et al. A novel mechanism for C1GALT1 in the regulation of gastric cancer progression. Cell Bioscience (2021) 11(1). doi: 10.1186/s13578-021-00678-2
82. Kuo TC, Wu MH, Yang SH, Chen ST, Hsu TW, Jhuang JY, et al. C1GALT1 high expression is associated with poor survival of patients with pancreatic ductal adenocarcinoma and promotes cell invasiveness through integrin αv. Oncogene (2021) 40(7):1242–54. doi: 10.1038/s41388-020-01594-4
83. Tan Z, Jiang Y, Liang L, Wu J, Cao L, Zhou X, et al. Dysregulation and prometastatic function of glycosyltransferase C1GALT1 modulated by cHP1BP3/ miR-1-3p axis in bladder cancer. J Exp Clin Cancer Res (2022) 41(1):228. doi: 10.1186/s13046-022-02438-7
84. Rømer TB, Khoder-Agha F, Aasted MKM, de Haan N, Horn S, Dylander A, et al. CRISPR-screen identifies ZIP9 and dysregulated Zn2+ homeostasis as a cause of cancer-associated changes in glycosylation. Glycobiology (2023) 33(9):700–14. doi: 10.1093/glycob/cwad003
85. Lee PC, Chen ST, Kuo TC, Lin TC, Lin MC, Huang J, et al. C1GALT1 is associated with poor survival and promotes soluble Ephrin A1-mediated cell migration through activation of EPHA2 in gastric cancer. Oncogene (2020) 39(13):2724–40. doi: 10.1038/s41388-020-1178-7
86. van Vliet SJ, van Liempt E, Saeland E, Aarnoudse CA, Appelmelk B, Irimura T, et al. Carbohydrate profiling reveals a distinctive role for the C-type lectin MGL in the recognition of helminth parasites and tumor antigens by dendritic cells. Int Immunol. (2005) 17:661–9. doi: 10.1093/intimm/dxh246
87. Saeland E, van Vliet SJ, Backstrom M, van den Berg VC, Geijtenbeek TB, Meijer GA, et al. The C-type lectin MGL expressed by dendritic cells detects glycan changes on MUC1 in colon carcinoma. Cancer Immunol Immunother. (2007) 56:1225–36. doi: 10.1007/s00262-006-0274-z
88. Zeng Y, Zhang Q, Zhang Y, Lu M, Liu Y, Zheng T, et al. MUC1 predicts colorectal cancer metastasis: A systematic review and meta-analysis of case controlled studies. PloS One. (2015) 10:e0138049. doi: 10.1371/journal.pone.0138049
89. Kvorjak M, Ahmed Y, Miller ML, Sriram R, Coronnello C, Hashash JG, et al. Cross-talk between colon cells and macrophages increases ST6GALNAC1 and MUC1-sTn expression in ulcerative colitis and colitis-associated colon cancer. Cancer Immunol Res. (2020) 8:167–78. doi: 10.1158/2326-6066.CIR-19-0514
90. Li W, Zhang N, Jin C, Long MD, Rajabi H, Yasumizu Y, et al. MUC1-C drives stemness in progression of colitis to colorectal cancer. JCI Insight. (2020) 5. doi: 10.1172/jci.insight.137112
91. Nath S, Mukherjee P. MUC1: a multifaceted oncoprotein with a key role in cancer progression. Trends Mol Med. (2014) 20:332–42. doi: 10.1016/j.molmed.2014.02.007
92. Oei AL, Moreno M, Verheijen RH, Sweep FC, Thomas CM, Massuger LF, et al. Induction of IgG antibodies to MUC1 and survival in patients with epithelial ovarian cancer. Int J Cancer. (2008) 123:1848–53. doi: 10.1002/ijc.23725
93. Li Z, Yang D, Guo T, Lin M. Advances in MUC1-mediated breast cancer immunotherapy. Biomolecules. (2022) 12. doi: 10.3390/biom12070952
94. Von Mensdorff-Pouilly S, Moreno M, Verheijen RH. Natural and induced humoral responses to MUC1. Cancers (Basel). (2011) 3:3073–103. doi: 10.3390/cancers3033073
95. Bhatia R, Gautam SK, Cannon A, Thompson C, Hall BR, Aithal A, et al. Cancer-associated mucins: role in immune modulation and metastasis. Cancer Metastasis Rev. (2019) 38:223–36. doi: 10.1007/s10555-018-09775-0
96. Chen SH, Dallas MR, Balzer EM, Konstantopoulos K. Mucin 16 is a functional selectin ligand on pancreatic cancer cells. FASEB J. (2012) 26:1349–59. doi: 10.1096/fj.11-195669
97. Chaturvedi P, Singh AP, Chakraborty S, Chauhan SC, Bafna S, Meza JL, et al. MUC4 mucin interacts with and stabilizes the HER2 oncoprotein in human pancreatic cancer cells. Cancer Res. (2008) 68:2065–70. doi: 10.1158/0008-5472.CAN-07-6041
98. Baldus SE, Monig SP, Hanisch FG, Zirbes TK, Flucke U, Oelert S, et al. Comparative evaluation of the prognostic value of MUC1, MUC2, sialyl-Lewis(a) and sialyl-Lewis(x) antigens in colorectal adenocarcinoma. Histopathology. (2002) 40:440–9. doi: 10.1046/j.1365-2559.2002.01389.x
99. Gendler SJ, Lancaster CA, Taylor-Papadimitriou J, Duhig T, Peat N, Burchell J, et al. Molecular cloning and expression of human tumor-associated polymorphic epithelial mucin. J Biol Chem. (1990) 265:15286–93. doi: 10.1016/S0021-9258(18)77254-2
100. Posey AD Jr., Schwab RD, Boesteanu AC, Steentoft C, Mandel U, Engels B, et al. Engineered CAR T cells targeting the cancer-associated tn-glycoform of the membrane mucin MUC1 control adenocarcinoma. Immunity. (2016) 44:1444–54. doi: 10.1016/j.immuni.2016.05.014
101. Dreau D, Moore LJ, Wu M, Roy LD, Dillion L, Porter T, et al. Combining the specific anti-MUC1 antibody TAB004 and lip-MSA-IL-2 limits pancreatic cancer progression in immune competent murine models of pancreatic ductal adenocarcinoma. Front Oncol. (2019) 9:330. doi: 10.3389/fonc.2019.00330
102. Supruniuk K, Czarnomysy R, Muszynska A, Radziejewska I. Anti-cancer effects of pyrazole-platinum(II) complexes combined with anti-MUC1 monoclonal antibody versus monotherapy in DLD-1 and HT-29 colon cancer cells. Transl Oncol. (2022) 18:101348. doi: 10.1016/j.tranon.2022.101348
103. Guo M, You C, Dong W, Luo B, Wu Y, Chen Y, et al. The surface dominant antigen MUC1 is required for colorectal cancer stem cell vaccine to exert anti-tumor efficacy. BioMed Pharmacother. (2020) 132:110804. doi: 10.1016/j.biopha.2020.110804
104. Ahmad R, Alam M, Hasegawa M, Uchida Y, Al-Obaid O, Kharbanda S, et al. Targeting MUC1-C inhibits the AKT-S6K1-elF4A pathway regulating TIGAR translation in colorectal cancer. Mol Cancer. (2017) 16:33. doi: 10.1186/s12943-017-0608-9
105. Sanz-Martinez I, Pereira S, Merino P, Corzana F, Hurtado-Guerrero R. Molecular recognition of galNAc in mucin-type O-Glycosylation. Acc Chem Res. (2023) 56:548–60. doi: 10.1021/acs.accounts.2c00723
Keywords: C1GALT1, core 1 β1-3 galactosyltransferase 1, T-synthase, O-glycosylation, colorectal cancer
Citation: Tian H, Yu J-L, Chu X, Guan Q, Liu J and Liu Y (2024) Unraveling the role of C1GALT1 in abnormal glycosylation and colorectal cancer progression. Front. Oncol. 14:1389713. doi: 10.3389/fonc.2024.1389713
Received: 22 February 2024; Accepted: 25 March 2024;
Published: 18 April 2024.
Edited by:
Ning Wei, Albert Einstein College of Medicine, United StatesReviewed by:
Liqun Yang, Shengjing Hospital of China Medical University, ChinaSuisui Hao, University of Southern California, United States
Copyright © 2024 Tian, Yu, Chu, Guan, Liu and Liu. This is an open-access article distributed under the terms of the Creative Commons Attribution License (CC BY). The use, distribution or reproduction in other forums is permitted, provided the original author(s) and the copyright owner(s) are credited and that the original publication in this journal is cited, in accordance with accepted academic practice. No use, distribution or reproduction is permitted which does not comply with these terms.
*Correspondence: Hong Tian, sysytianhong@163.com
†These authors have contributed equally to this work and share first authorship